Organ-on-a-chip technology: innovations, applications, and future horizons
Organ-on-a-chip technology emerged in the early 21st century as a revolutionary approach to simulate human organ functions in vitro. So, what is an organ-on-a-chip? What are its main applications and advantages? And to what extent is it a promising tool for drug testing and disease modeling? We answer all these questions in the following review.
Introduction to organ-on-a-chip
An organ-on-a-chip is a microengineered cell culture device that mimics the functions of human organs. This innovation was driven by the limitations of traditional cell culture methods and animal testing, which often failed to replicate human physiology accurately. Organ-on-a-chip has been increasingly used, improving the translational value of several research fields, including drug screening, tissue engineering, and more.
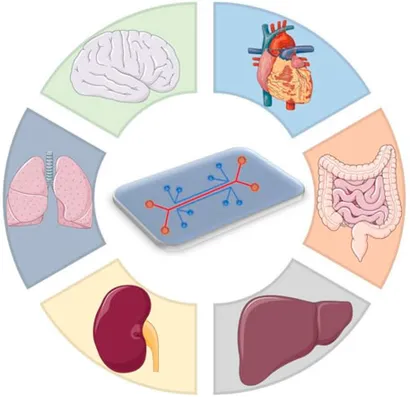
This review provides an overview of organ-on-a-chip technology, its applications, its benefits compared to conventional models, and its manufacturing techniques. We will also examine the different organ models and the future of organ-on-a-chip technology.
Organ-on-a-chip and microfluidics
Organ-on-a-chip (OoC) are cell cultures, often in 3D, that use microfluidics to reproduce how a tissue or part of an organ works (Figure 1). The field gained momentum with advancements in microfabrication and tissue engineering, allowing researchers to create microfluidic devices that replicate the functions of living organs by mimicking their microstructures, dynamic mechanical properties, and biochemical functionalities [1]. These devices integrate microfluidics, cell biology, bioengineering, and biomaterial technology, making them valuable tools for tissue engineering, disease modeling, and preclinical drug testing [2].
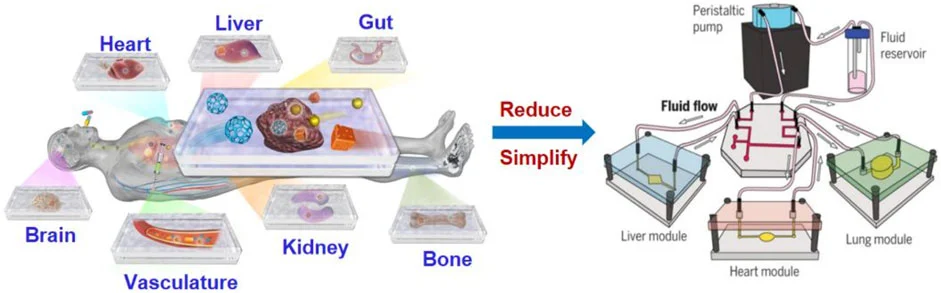
Why is organ-on-a-chip technology so important today?
The need for organ-on-a-chip models
Pharmaceutical companies are investing more in clinical trials but are introducing few new therapies. Current preclinical models cannot fully recapitulate human organs’ complex nature and function, leading to limited accuracy and poor predictability in drug development [4] (Figure 2). For decades, traditional two-dimensional (2D) cell culture systems constituted a vital platform to study the functions of various cells. However, 2D systems fail to accurately simulate the physiological manifestations of living tissues/organs, intra-organ interactions, and microenvironmental factors [5] and often require validation in in vivo animal models.
Preclinical animal models have been essential for disease modeling and drug development, but they struggle to accurately predict human responses due to differences in species genetics. In addition, the use of animal models has come under scrutiny due to both high costs and ethical issues [6]. Moreover, only a fraction of potential drug candidates from extensive molecular libraries can be thoroughly assessed. Furthermore, only one out of nine drug candidates (11%) that enter the clinical study phase is successfully introduced into the market due to a lack of efficacy and safety in humans, even though animal testing predicts otherwise [7].
It was time to find a solution to accelerate research. Organ-on-a-chip was proposed as a future replacement technology for experimental animal models [8]. It was designed to overcome the shortcomings mentioned above by:
- Creating a complex tissue-like environment with several cell types
- Mimicking different organ areas (ex, bronchi, alveoli, etc.…in the case of lungs)
- Reflecting physiological/diseased mechanical cues at cellular and tissue levels
- Providing a controlled and dynamic microenvironment
- Rapidly testing drugs/compounds with high-throughput outcomes
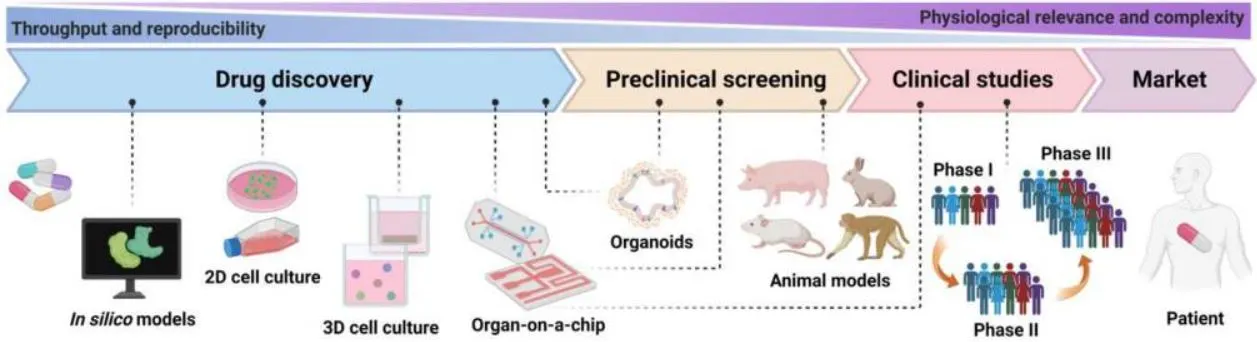
Organ-on-a-chip is mainly used for tissue engineering, disease modeling, and drug screening (Figure 3).
Tissue engineering
Organ-on-a-chip devices replicate organ-level structures (tissue architecture, tissue-tissue interfaces), mechanical properties, and biochemical cues, providing a more accurate representation of organ physiology. Microfluidics combined with organ-on-a-chip enables real-time microenvironment control (nutrient and gas exchange, molecular gradients), fluid flow, and dynamic mechanical forces (blood flow, breathing, or peristalsis-like motions), where the fluid/gas flow is accurately regulated by flow control systems, and cellular responses monitored via integrated sensors [10].
Complex disease modeling
Organ-on-a-chip systems can recreate organism-level functions and whole-body physiology by fluidically coupling two or more OoC (human-on-a-chip model). The latter is essential to study diseases affecting multiple organs or systems, such as diabetes and metastatic cancers. In addition, organ-on-a-chip platforms enable long-term studies of chronic diseases by maintaining viable cell cultures over extended periods [2].
Drug screening and toxicity testing
Microfluidic chips embedded in organ-on-a-chip platforms facilitate the generation of biochemical gradients and concentration, allowing researchers to study cellular responses to drug compounds with unprecedented detail. By accurately replicating human tissue and organ functions, organ-on-a-chip constitutes a more reliable platform for investigating new drugs’ mechanism of action, efficacy, and potential side effects [11]. Thus, organ-on-a-chip technology enhances the assessment of drug-induced toxicity and improves the efficiency of the candidate drug selection process [12]. Thereby, the drug development process is streamlined, and reliance on animal models is reduced.
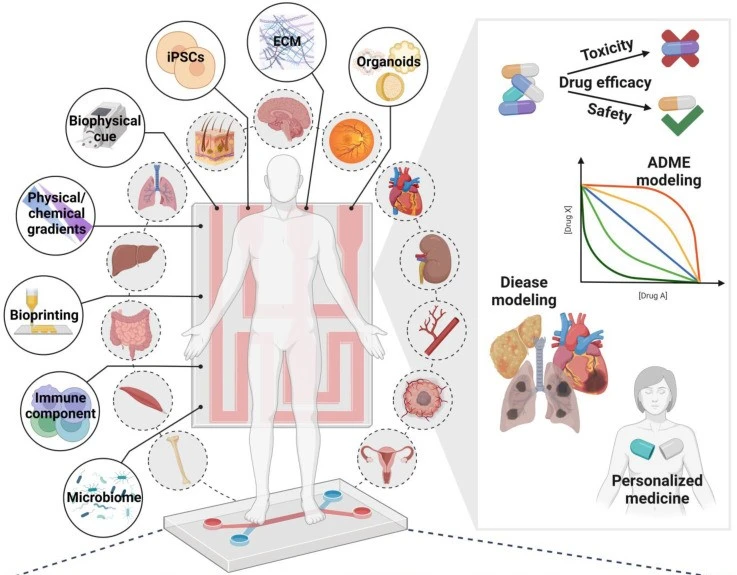
What techniques and types of chips are used to fabricate organ-on-a-chip models?
Fabrication of organ-on-a-chip models
Fabrication techniques
Several techniques are employed to manufacture microfluidic chips used in organ-on-a-chip systems. The fabrication method and material are selected according to the study’s purpose [13].
Soft lithography
Soft lithography is a process where a microfluidic chip layout is engraved in a soft material, such as polydimethylsiloxane (PDMS) (Figure 4a). PDMS is suitable for fabricating a few micrometer-scaled microchannels. In addition, soft lithography offers advantages such as ease of fabrication (fast prototyping), easy handling, integration of different layers and membranes, and the ability to adapt the architecture easily to experiment needs.
In addition, PDMS chips are transparent, which makes them suitable for organ-on-a-chip cultures. However, PDMS is permeable to air (oxygen and CO2), it can dry out, and it can absorb small and hydrophobic molecules, which hinders the use of PDMS-based OoC in drug studies, as the actual dose of the introduced drug will not be clear due to the absorbance effect [13].
Injection molding
Injection molding (Figure 4b) is another fabrication technique widely used in commercialized OoC systems because it allows for mass production [14]. Injection-molded materials, mostly thermoplastics, such as polymethyl methacrylate (PMMA), cyclic olefin copolymer (COC), polystyrene (PS), or polycarbonate (PC), have gained interest as an alternative to conventional materials.
These materials present better mechanical properties than PDMS and are more robust and accessible to manufacture than traditional materials such as silicon (Si) or glass. In addition, thermoplastics offer optical transparency and compatibility with microfluidic applications. However, they are rigid and do not absorb or permeate other molecules.
3D printing techniques
Another fabrication technique is 3D printing, which is suitable for fabricating intricate microchannel networks, for example, by mimicking complex 3D human organ architecture. It offers precise control over the microchannel geometry [15]. Fabricating circular microchannels via soft lithography is challenging because the photoresist can only be crosslinked with a fixed height, whereas 3D printing techniques can control the spatial distribution via layer-by-layer assembly of the material [16].
Printing ink can be made of natural (bio-compatible) or synthetic materials, and various printing methods can be employed (micro-extrusion, inkjet, or laser-assisted printing) (Figure 4c). Laser-assisted techniques achieve the best spatial resolution, but inkjet printing allows cells to be printed together with their supporting matrix [13]. Notably, the 3D printing technique is also applicable for commercialized products and large-scale production of organ-on-a-chip.
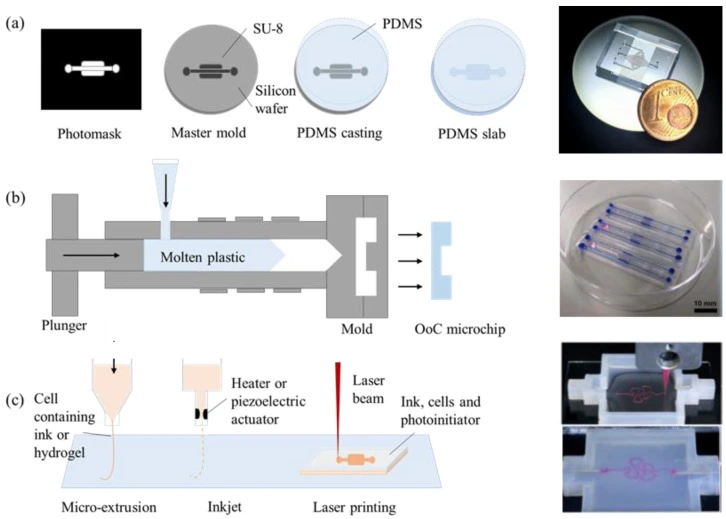
Examples of commercially available chips
Instead of fabricating chips from scratch, many researchers opt for prefabricated chips available online. Commercially available chips have advantages such as high reproducibility, industry-level quality control, and customer assistance. They usually have the standard format of a microscope slide or a multiwell place and are compatible with various microscopic techniques. Some examples are given below, highlighting products commonly used in organ-on-chip research.
Microfluidic ChipShop: The Barrier model chip
A cross-flow membrane chip, the Fluidic 480, was developed by microfluidic ChipShop GmbH (Figure 5). It serves for organ-on-a-chip barrier models of molecular transport across an interface. The chip has two stacked chambers interconnected by a semipermeable membrane. There are two units per slide. The chip is intended to be seeded with cells on either side of the central porous membrane to create an interfacial barrier layer from multiple cell types. Flow can be added to the upper, lower and/or both chambers, depending on the assay needs and sensitivity of the used cells.
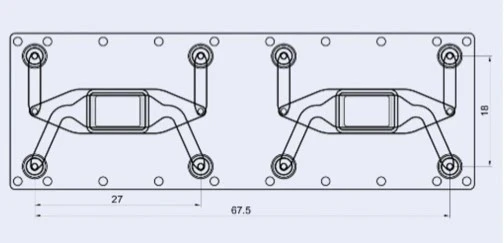
This chip was used in one of our experimental setups for microfluidic cell culture Cells were seeded in the upper chamber of the cross-flow membrane chip and perfused for 16 h. No flow was set in the lower channel. Cells were imaged at two positions: from below the membrane with the chip upright (position indicated by blue circle) or on the polymer surface (i.e., off the membrane; purple circle) (Figure 6).
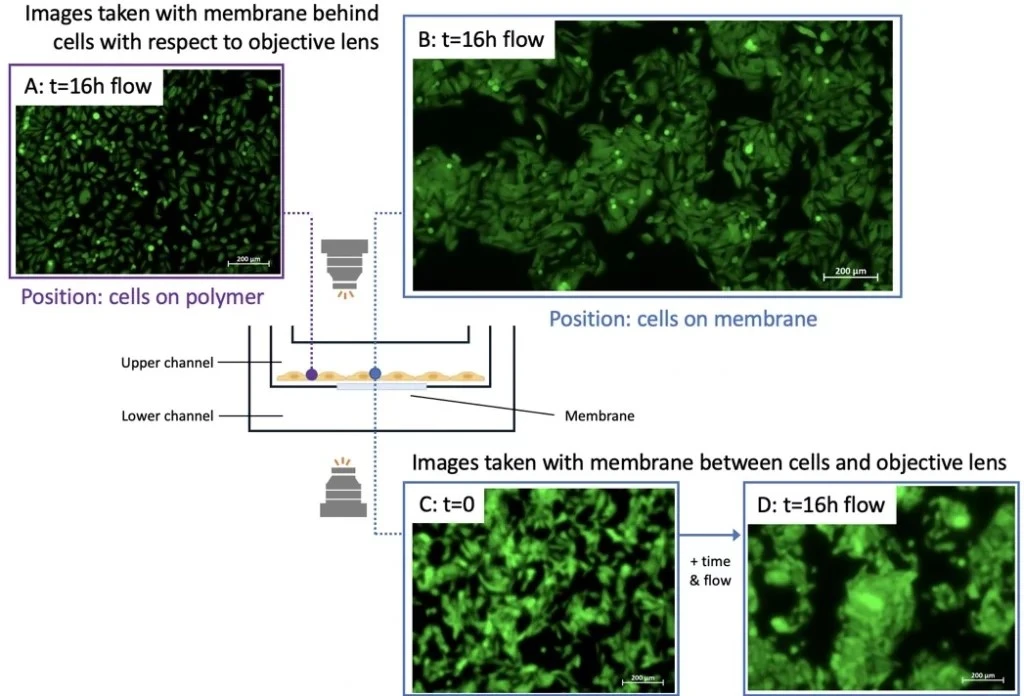
IVTech: Low-shear stress chips
IVTech MultiDyn bioreactor is a compact multi-dynamic-chamber rack based on the dimensions of a standard 24-well plate (Figure 7). Each rack is transparent and equipped with four wells that can be autonomously perfused, allowing for the development of 4 parallel and dynamic models.
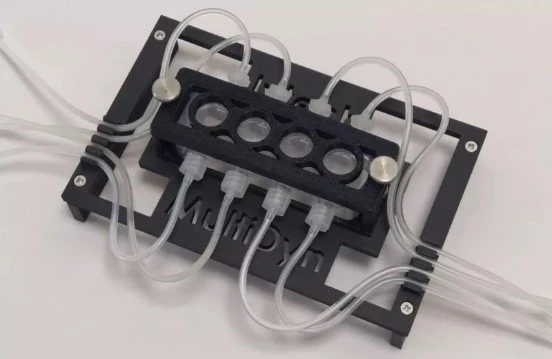
Barra and colleagues successfully developed a blood-brain barrier model using the bioreactor that ensured low shear stress and the medium’s circulation via continuous laminar flow. This dynamic model consisted of a double-culture chamber separated by a membrane on which brain endothelial cells are cultured to evaluate drug passage [17]. “Immunofluorescence experiments showed that fluid dynamic conditions have no appreciable influence on spheroids health, morphology, and organization” (Figure 8).
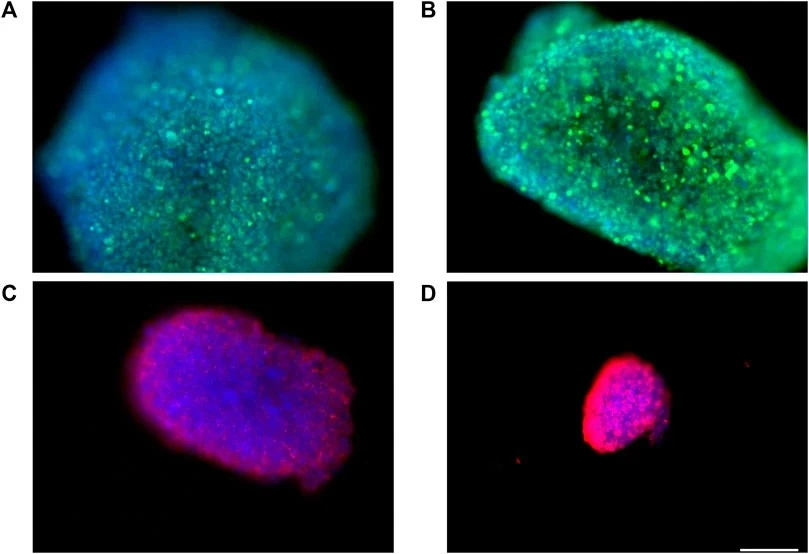
IBIDI: The µ-Slide product range
The µ-Slide III 3D Perfusion, developed by Ibidi company, is a three-channel µ-slide used to cultivate and perfuse 3D cell structures (Figure 9).
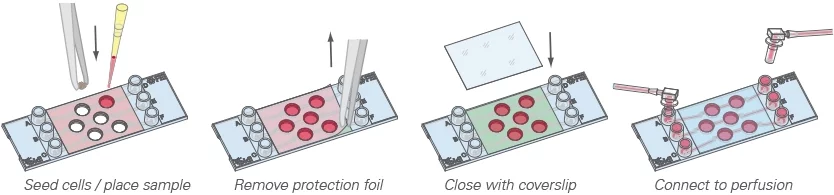
We used our stage-top incubator and the µ-Slide III 3D Perfusion to culture primary mouse microglial cells in static conditions for 48h of stimulation with lipopolysaccharide (LPS) (Figure 10a) and HEK293 cells under flow on the microscope stage for 60 hours (Figure 10b).
In the latter experiment, the gas permeability of the chips was assessed to understand the influence of lack of CO2 control on cell culture outside the CO2 incubator. Cells grew and multiplied in both conditions, with the impenetrable chip showing more considerable pH variations without negatively impacting cell doubling (Figure 10b).
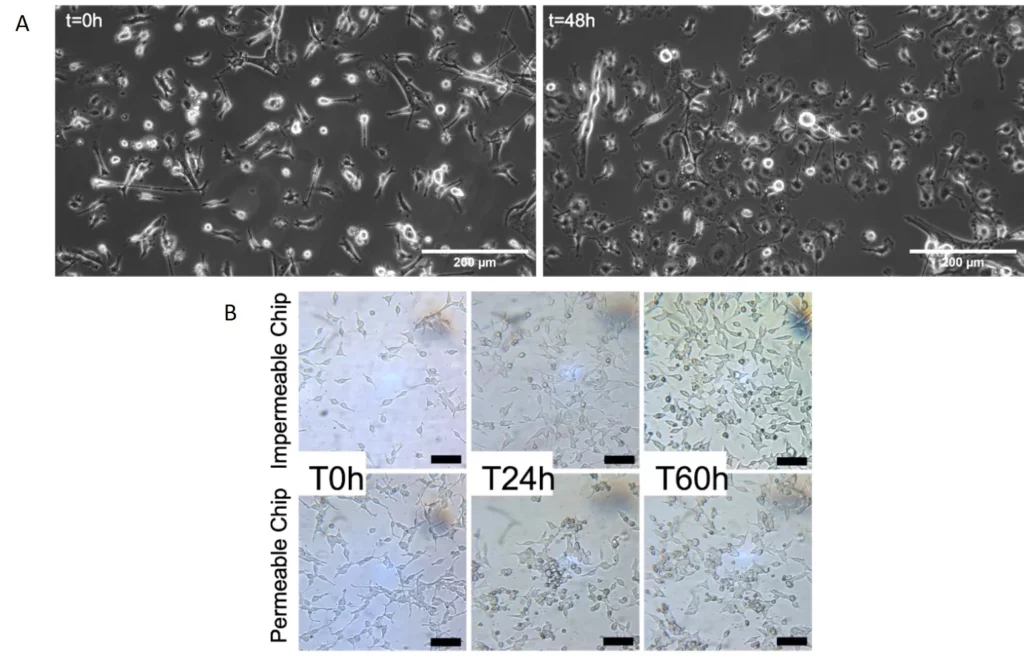
Cherry Biotech: Examples of a multiwell configuration
The CubiX platform, developed by Cherry Biotech, includes a control unit (software, gas & flow controller), a heating stage, perfusion lid(s), and a 24-well chip. It can culture differentiated endothelium, high-throughput organoids, fully differentiated vascularized and immunocompetent skin, blood-brain barrier, and 3D liver cells.
By closing the perfusion lid, the standard multiwell configuration can be transformed into a dynamic perfusion system (Figure 11).
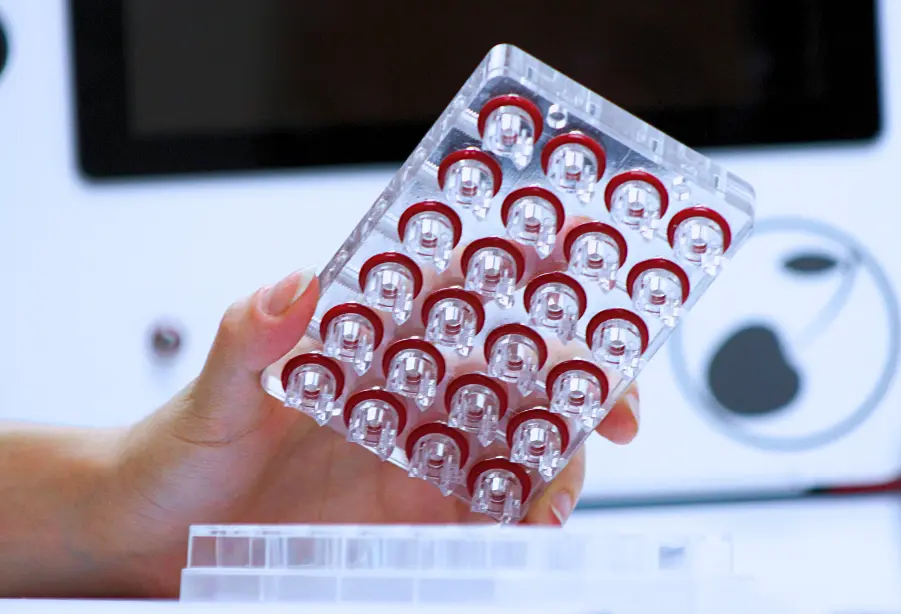
What organ models have been developed using organ-on-a-chip technology?
Organ models
Several organ-on-a-chip devices have been successfully developed. They can be single-organ (lung, gut, liver, kidney, blood vessels, bone, skin-on-a-chip), modeling specific body systems and disease states (blood-brain barrier, tumor-on-chip), or multi-organ (model connecting liver, heart, bone, and skin).
Single-organ-models
Lung-on-a-chip
The lung-on-chip model was one of the first milestones of OoC technologies, with the “breathing lung” developed in 2010 by Huh and colleagues. The researchers created a biomimetic device designed to miniaturize the lung alveolar-capillary interface by replicating its structural, functional, and mechanical characteristics (Figure 12) [18]. This device consisted of two PDMS microchannels separated by a porous PDMS membrane, with human alveolar epithelial cells on one side and pulmonary endothelial cells on the other. By injecting air, they successfully simulated the human breathing process at the alveolar interface.
The lung-on-a-chip model is instrumental in studying respiratory diseases such as asthma, thrombosis, chronic obstructive pulmonary disease, and pulmonary fibrosis. By exposing the lung model to environmental toxins, pathogens, and drug compounds, researchers can elucidate disease pathogenesis and evaluate therapeutic interventions.
The lung-on-a-chip model can be developed using our lung-on-a-chip pack. Endothelial cells can be cultured on a chip using the endothelial cell culture pack.
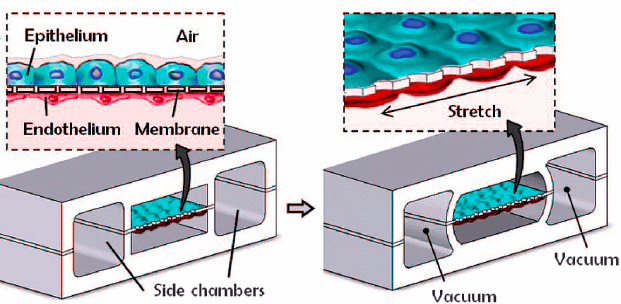
Gut-on-a-chip
The most common gut-on-a-chip model structure has upper and lower channels separated by a porous semipermeable membrane, which depicts the separation between the intestinal lumen and the vasculature (Figure 13). The semipermeable membrane facilitates the transport of soluble molecules and nutrients between the gut and the blood vessels [19].
Kasendra and colleagues developed a bioengineered in vitro model of the duodenum of the small intestine by combining microfluidic organ-on-a-chip technology with patient biopsy-derived duodenal organoids. The chip recapitulates important structural features of the native duodenum, including the formation of elongated villi-like structures, enteroendocrine cells, basal proliferative cells, strong barrier function, digestive enzyme activity, and continuous accessibility to fluids flowing through the apical lumen [20].
A gut-on-a-chip model can be developed using our gut-on-a-chip pack
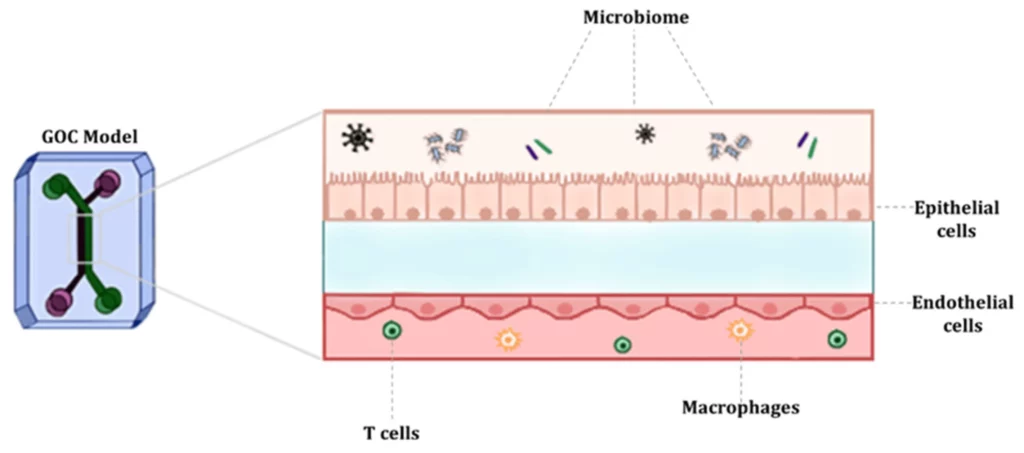
Liver-on-a-chip
The liver-on-a-chip model incorporates hepatocytes, Kupffer cells, stellate cells, endothelial cells, or human pluripotent stem cell derivatives, recapitulating hepatic functions and interactions. Weng and colleagues developed a liver-on-a-chip device without scaffolding (Figure 14). Their idea was to bypass the need for a scaffold by biomimicking the reconstruction of tissue hierarchy with the introduction of primary hepatic stellate cells (HSCs) to incorporate physiologically relevant extracellular matrix. Controlled flow was introduced to mimic the flow from the portal vein to the central vein and the radial flow in the liver lobule [21].
Liver-on-a-chip is a valuable tool for studying liver diseases, including hepatitis, fatty liver disease, and drug-induced liver injury. This model can be developed using our liver-on-a-chip pack.
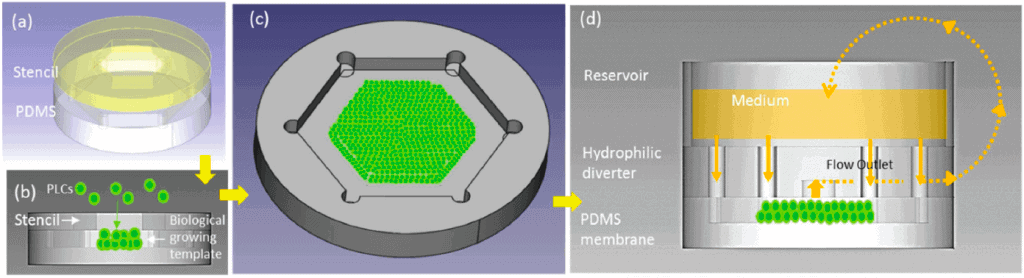
Kidney-on-a-chip
The kidney-on-a-chip model consists of renal epithelial, endothelial, and immune cells, resembling the nephron structure and function. The first model was reported in 2007, describing a distal tubule dynamic model for toxicity testing based on canine kidney cells [22].
Other areas of the kidney, such as proximal tubules [23], the glomerulus [24], and the nephron [25], have been reproduced on-chip. By simulating glomerular filtration (glomerulus-on-a-chip), tubular reabsorption (proximal tubule-on-a-chip), and interstitial inflammation, kidney models enable the study of disease mechanisms and the screening of potential therapeutics.
The glomerulus-on-a-chip model is the most common kidney model. Glomerular endothelial cells (GECs) are implanted into the upper vascular channel, while podocytes are placed in the lower urinary channel (Figure 15). A porous and flexible membrane separates the two channels, mimicking the three-layer structure of the glomerular filtration barrier and simulating hemofiltration as blood and urine flow through the microdevice [24].
Kidney-on-a-chip models can be developed using our kidney-on-a-chip pack.
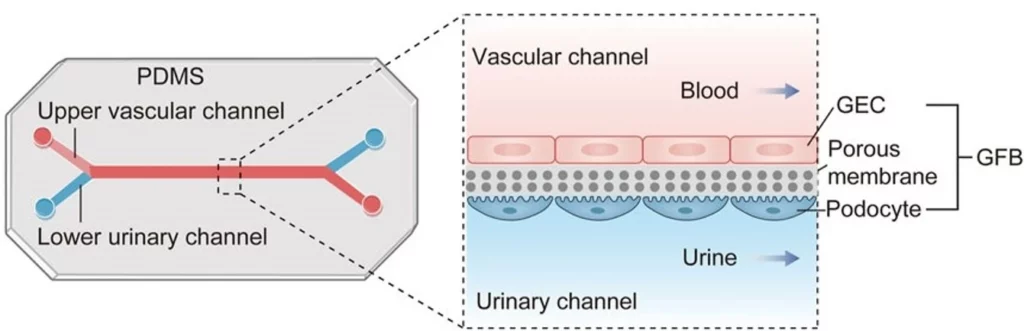
Heart-on-a-chip
The heart-on-a-chip model incorporates cardiomyocytes, fibroblasts, endothelial cells, and cardiac progenitor cells, replicating the myocardial tissue architecture.
Abulaiti and colleagues reported a new bioassay platform based on human-induced pluripotent stem cell (iPSC) technology and a microelectromechanical system (MEMS) that evaluates physiological heart function (Figure 16). The platform showed a significant correlation with human heart physiology. It serves as a tool for “order-made” cardiac toxicity tests since it can be prepared with heart disease-specific human iPSCs for the discovery of disease-specific treatment drugs for which the cardiac toxicity varies according to individual biological backgrounds [26].
We have designed a pack to develop blood-vessel-on-a-chip.
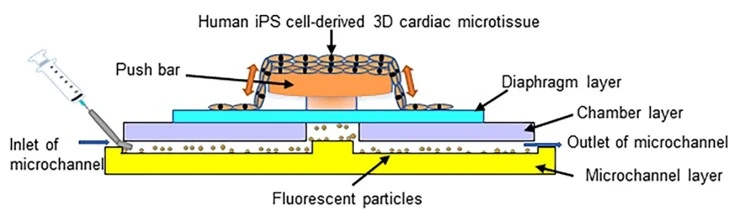
Bone-on-a-chip
The bone is one of the most complex organs in the body. It operates in a fine balance between osteocytes and osteoclasts, with the former synthesizing new bone and the latter resorbing it.
To investigate osteogenesis, Bahmaee and colleagues designed a two-part microfluidic device consisting of a bioreactor and a 3D bone tissue engineering scaffold (Figure 17) and cultured human embryonic stem cell-derived mesenchymal progenitor cells (hES-MPs) for three weeks. They tested different flow rates and profiles to assess metabolic activity, osteogenic differentiation, and mineralized matrix deposition. An intermittent flow profile was shown to promote cell differentiation and enhance mineralized matrix deposition [27].
We assembled a pack to create bone-on-a-chip models.
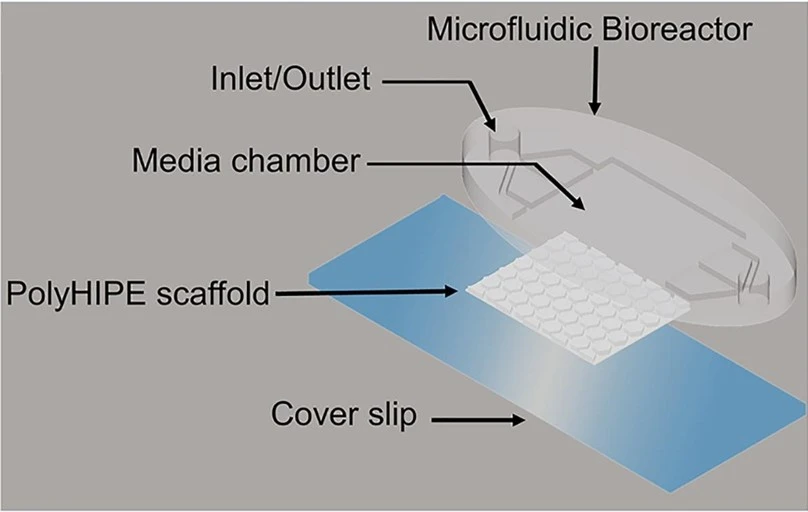
Skin-on-a-chip
The skin-on-a-chip model has three layers: epidermal, dermal, and vascular. Each layer is separated using transparent, porous membranes to allow interlayer communication and mimic skin biology. It is mostly used to study infectious skin diseases.
Sun and colleagues created a biologically relevant platform for studying human simplex virus (HSV)- infected skin (Figure 18). This model, incorporating donated skin tissue and vascularized microfluidic channels, enabled the simulation of tissue microinjury, facilitating virus entry and the introduction of HSV and human neutrophils [28].
Kim and colleagues incubated a human skin equivalent (HSE) acquired through skin donation in varying bacterial concentrations to observe neutrophil responses to Staphylococcus aureus infections. Their findings affirmed neutrophil migration as a diagnostic biomarker for skin infections. Furthermore, the model was proven efficient in assessing broad-spectrum antibiotic treatments and individualized dosages based on infection burden [29].
We have assembled a pack to develop skin-on-a-chip models.
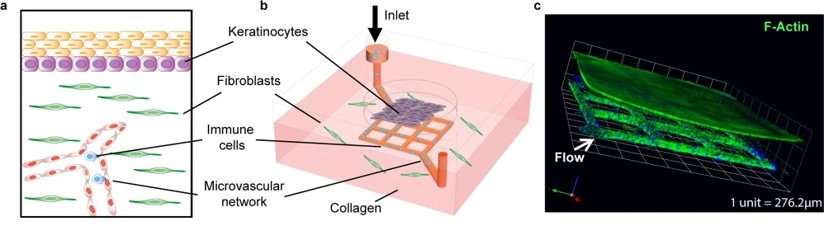
Models for specific body systems and disease states
Tumor-on-a-chip
In the Microfluidics Innovation Center, we have developed a lymph node model pack in collaboration with our partners at the National Technical University of Athens NTUA to study lung metastasis.
Culturing cells under flow improves the physiological relevance of fluid-dynamic organs such as the lymph nodes. In addition, the lymph node model includes a cell culture pump, which maintains a constant gas concentration and optimal pH for long-term cell culture outside the CO2 incubator. The stage-top incubator maintains the temperature at 37°C and allows you to leave your cells on top of the microscope stage and perform higher-quality live cell imaging.
Our partners from the Tumor-LN-oC project cultured Lewis lung carcinoma (LLC) cells under flow in the project-specific chip using our lymph node model pack (Figure 19a). The culture was terminated after three days to test the cells’ viability. After the successful results of the 3-day culture, the experiment was repeated (Figure 19b, c, e). The culture was viable for up to 16 days (Figure 19d).
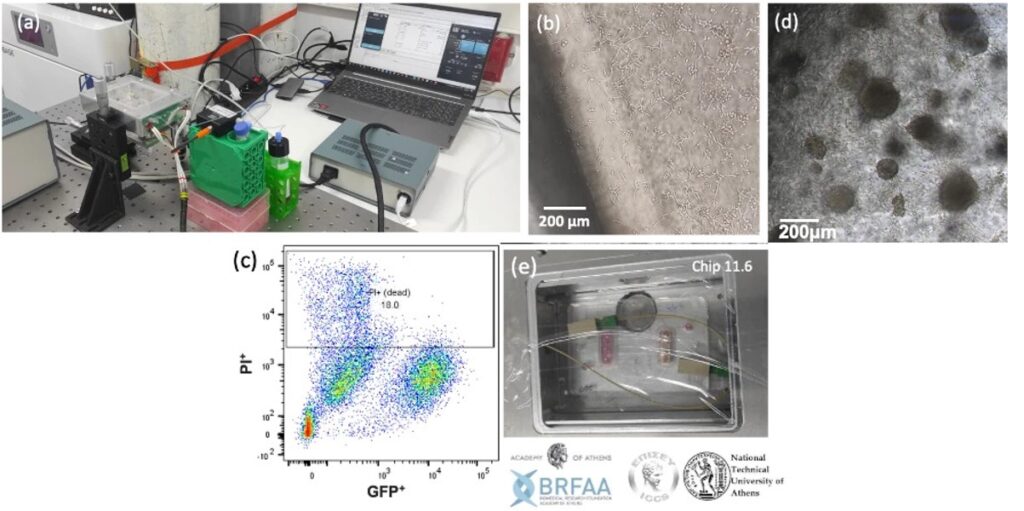
Blood-brain-barrier-on-a-chip
The blood-brain barrier (BBB) is a key barrier to delivering many neuroactive therapeutics and is central to many neurological diseases. The semi-permeability of the endothelial barrier is needed to maintain brain homeostasis without allowing neurotoxic molecules to enter; however, many therapeutic drugs are also blocked.
Many human BBB chip models of the neurovascular unit have been developed. The chip contains a brain endothelium interfaced with either brain pericytes and astrocytes or astrocytes and neurons; some are lined with one or more cell types derived from iPSCs [30] (Figure 20). Human BBB models have also been used to model neuroinflammation [30], protection of neurons from injury, and shuttling of drugs, hormones, monoclonal antibodies, nanocarriers, and tumor extracellular vesicles across the BBB [31].
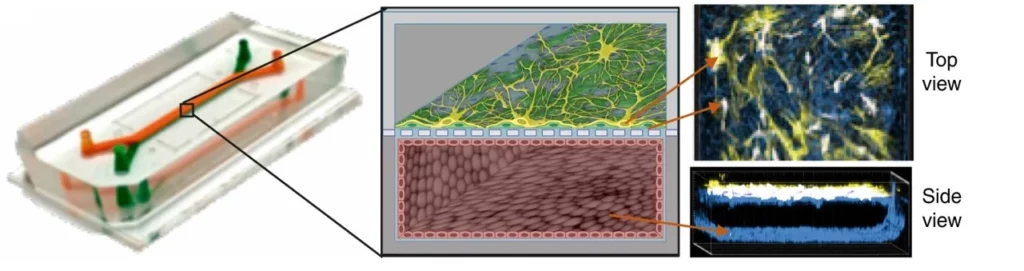
Multi-organs-on-chip - Body-on-a-chip
Multi-organs-on-chip have emerged as miniature replicas of the human body, often consisting of two or more coupled organ-on-a-chip platforms. The goal is to link a maximum number of OoCs to reproduce a body-on-a-chip (BoC). Human BoC requires the recirculation of a commonly shared medium that enables reciprocal communication between organ components while allowing them to maintain their own identity [32]. It could also allow us to witness the side effects of certain drugs on different organs, not just those that the treatment targets.
Recently, Ronaldson-Bouchard and colleagues developed a BoC housing 3D-engineered heart, liver, bone, and skin fluidically connected via a shared simulated vascular flow (Figure 21). Most tissue components in the BoC were engineered with iPSC-derived cells seeded in different biomaterials. The device contained four tissue chambers, a perfusate flow channel, and a medium reservoir. The model was validated to characterize the transfer of cardiomyocyte secretome to different tissues and investigate exosome-mediated communication between the heart and other organs [33].

What challenges are currently faced by organ-on-a-chip technology, and what potential does this technique hold for the future?
Considerations and future directions
Despite the advances made in organ-on-a-chip systems, recreating the complex, multifaceted integration of intricate structural and functional features of native organs in BoCs is challenging. First, a modular, “plug-and-play” design is highly desirable to conveniently integrate different organ modules (Figure 22 a).
In addition, the heterogeneity of many human diseases requires individualized investigation. In the long run, organs-on-chip could allow everyone access to individualized treatments by testing them using their cells. A personalized “patient-on-a-chip” can be generated using patient-specific iPSCs reprogrammed from somatic cells (Figure 22 b). Thus, effective and standardized protocols for the induction of iPSC differentiation and maturation into specific tissue lineages are warranted [34].
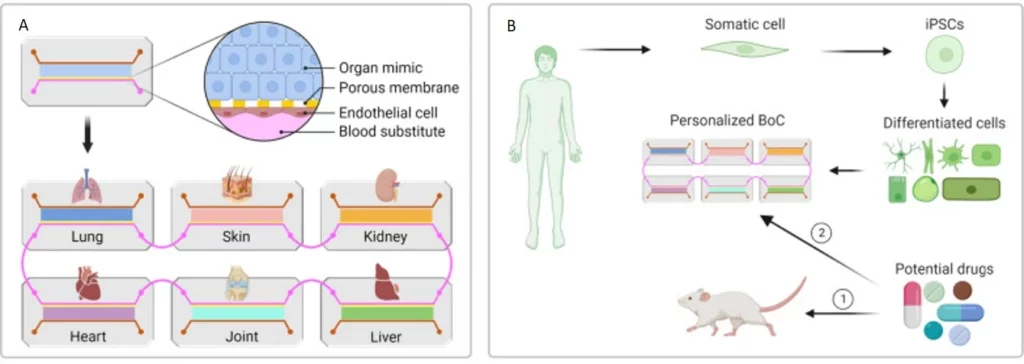
Notably, previous BoC platforms didn’t accurately address the need to downscale human body components. For instance, the number and density of tissue-specific cells and medium volume should reflect the relative abundance and distribution in the native organs. Moreover, it is critical to validate results obtained from OoCs with data obtained from human patients [32]. Finally, most organ-on-a-chip systems are routinely subjected to higher oxygen levels than their native counterparts, a limitation that needs to be overcome in future designs [32].
Despite the challenges facing organ-on-a-chip models, technological advancements in robotics, sensors, and artificial intelligence (AI) are promising as they can simplify handling, automate monitoring, and facilitate analysis.
Conclusion - Towards complex BoC systems
Microfluidics integrated into organ-on-a-chip added a precise and controlled flow, introducing a hallmark of the native cell environment currently missing from most static organ-on-a-chip. OoC platforms, combining microfabrication techniques, advanced cell culture methods, and innovative sensing technologies, recapitulate the complexities of human organs with remarkable fidelity. Engineered patient-specific cell-derived BoC can assess the safety and efficacy of personalized treatments and potentially predict those that “fail fast” in preclinical tests, enhancing the drug discovery rate. Moreover, the continuous progress in robotics, sensors, and AI is promising. For example, liquid-handling robotics can enable the fluidic coupling of many OoC modules, establishing complex BoC systems with high physiological relevance. Thus, organ-on-a-chip systems will dramatically change drug development, disease modeling, and personalized medicine.
Funding and Support
This review was written under the European Union’s H2020-NMBP-TR-IND-2020, grant agreement No. 953234 (Tumor-LN-oC),
the European Union’s H2020-LC-GD-2020-3 grant agreements No. 101036702 (LIFESAVER) and 101037090 (ALTERNATIVE),
and the French National Research Agency (ANR) & the German Federal Ministry of Education and Research (BMBF) in the frame of Locai project.
This review was written by Celeste Chidiac, PhD.
Published in August 2024.
Contact: Partnership[at]microfluidic.fr
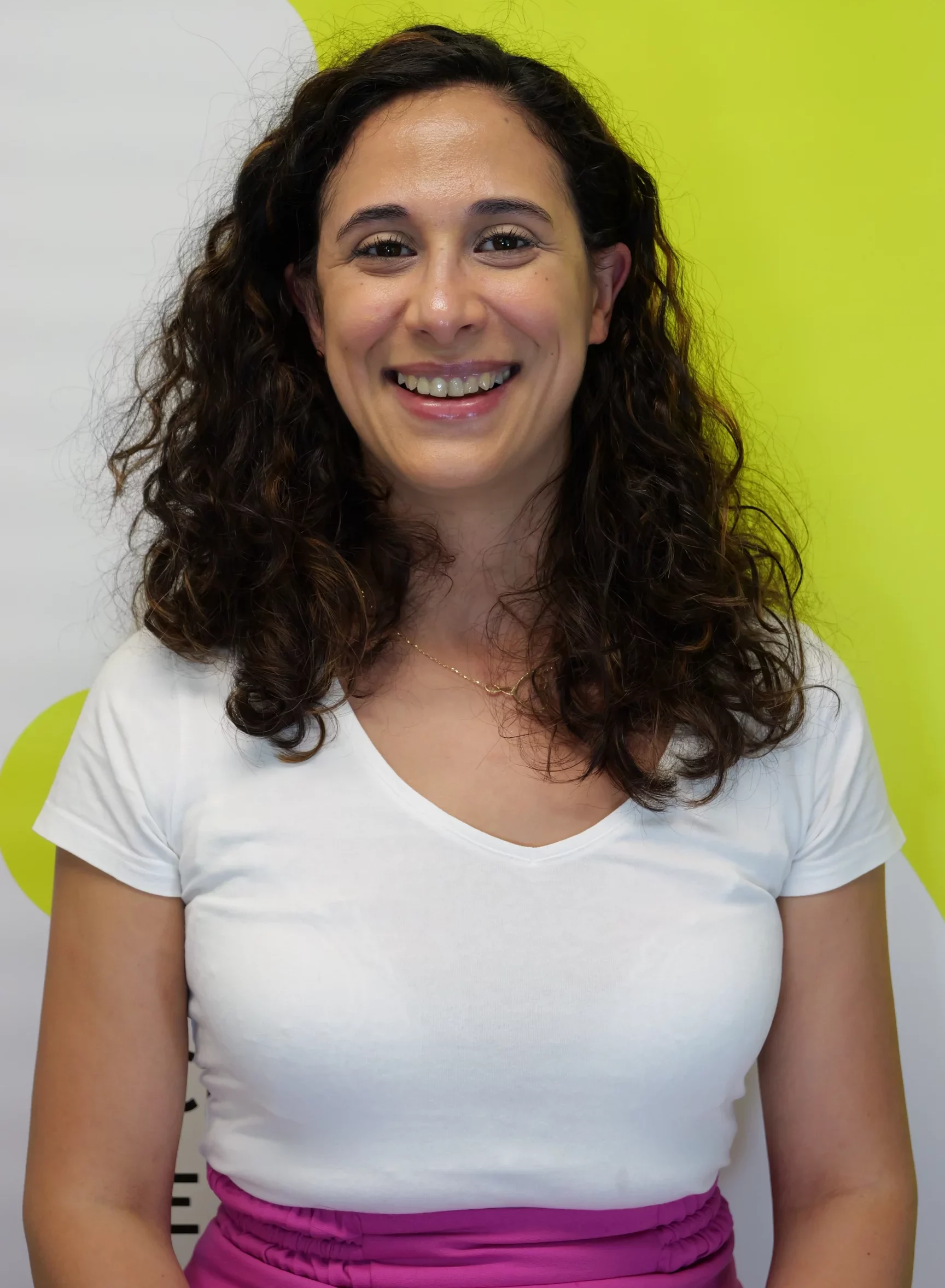
References
- Wu, Q., et al., Organ-on-a-chip: recent breakthroughs and future prospects. Biomed Eng Online, 2020. 19(1): p. 9.
- Ingber, D.E., Human organs-on-chips for disease modelling, drug development and personalized medicine. Nature Reviews Genetics, 2022. 23(8): p. 467-491.
- Yang, Y., et al., PBPK Modeling on Organs-on-Chips: An Overview of Recent Advancements. Frontiers in Bioengineering and Biotechnology, 2022. 10: p. 900481.
- Halldorsson, S., et al., Advantages and challenges of microfluidic cell culture in polydimethylsiloxane devices. Biosens Bioelectron, 2015. 63: p. 218-231.
- Jiang, K., et al., Microfluidic-based biomimetic models for life science research. RSC advances, 2016. 6(32): p. 26863-26873.
- Al-Lamki, R.S., J.R. Bradley, and J.S. Pober, Human Organ Culture: Updating the Approach to Bridge the Gap from In Vitro to In Vivo in Inflammation, Cancer, and Stem Cell Biology. Front Med (Lausanne), 2017. 4: p. 148.
- van Berlo, D., et al., The potential of multi-organ-on-chip models for assessment of drug disposition as alternative to animal testing. Current Opinion in Toxicology, 2021. 27: p. 8-17.
- Reardon, S., ‘Organs-on-chips’ go mainstream. Nature, 2015. 523(7560): p. 266.
- Deng, S., et al., Organ-on-a-chip meets artificial intelligence in drug evaluation. Theranostics, 2023. 13(13): p. 4526-4558.
- Mandrycky, C.J., et al., Organ-on-a-chip systems for vascular biology. J Mol Cell Cardiol, 2021. 159: p. 1-13.
- Phan, D.T.T., et al., A vascularized and perfused organ-on-a-chip platform for large-scale drug screening applications. Lab Chip, 2017. 17(3): p. 511-520.
- Rajan, S.A.P., et al., Probing prodrug metabolism and reciprocal toxicity with an integrated and humanized multi-tissue organ-on-a-chip platform. Acta Biomaterialia, 2020. 106: p. 124-135.
- Ko, J., et al., Engineering Organ-on-a-Chip to Accelerate Translational Research. Micromachines (Basel), 2022. 13(8).
- Virumbrales-Muñoz, M., et al., Enabling cell recovery from 3D cell culture microfluidic devices for tumour microenvironment biomarker profiling. Sci Rep, 2019. 9(1): p. 6199.
- Au, A.K., et al., 3D-Printed Microfluidics. Angewandte Chemie International Edition, 2016. 55(12): p. 3862-3881.
- Lee, B., et al., 3D micromesh-based hybrid bioprinting: multidimensional liquid patterning for 3D microtissue engineering. NPG Asia Materials, 2022. 14(1): p. 6.
- Barra, T., et al., gH625-liposomes deliver PACAP through a dynamic in vitro model of the blood-brain barrier. Front Physiol, 2022. 13: p. 932099.
- Huh, D., et al., Reconstituting Organ-Level Lung Functions on a Chip. Science, 2010. 328(5986): p. 1662-1668.
- Thomas, D.P., et al., Microfluidic Gut-on-a-Chip: Fundamentals and Challenges. Biosensors, 2023. 13(1): p. 136.
- Kasendra, M., et al., Development of a primary human Small Intestine-on-a-Chip using biopsy-derived organoids. Scientific Reports, 2018. 8(1): p. 2871.
- Weng, Y.-S., et al., Scaffold-Free Liver-On-A-Chip with Multiscale Organotypic Cultures. Advanced materials (Deerfield Beach, Fla.), 2017. 29(36).
- Baudoin, R., et al., Development of a renal microchip for in vitro distal tubule models. Biotechnology progress, 2007. 23(5): p. 1245-1253.
- Ng, C.P., et al., A Fibrin-Based Tissue-Engineered Renal Proximal Tubule for Bioartificial Kidney Devices: Development, Characterization and In Vitro Transport Study. International Journal of Tissue Engineering, 2013. 2013.
- Zhou, M., et al., Development of a Functional Glomerulus at the Organ Level on a Chip to Mimic Hypertensive Nephropathy. Scientific Reports, 2016. 6(1): p. 31771.
- Weinberg, E., M. Kaazempur-Mofrad, and J. Borenstein, Concept and computational design for a bioartificial nephron-on-a-chip. Int J Artif Organs, 2008. 31(6): p. 508-14.
- Huang, W., et al., Revolutionizing nephrology research: expanding horizons with kidney-on-a-chip and beyond. Front Bioeng Biotechnol, 2024. 12: p. 1373386.
- Abulaiti, M., et al., Establishment of a heart-on-a-chip microdevice based on human iPS cells for the evaluation of human heart tissue function. Scientific Reports, 2020. 10(1): p. 19201.
- Pediaditakis, I., et al., Modeling alpha-synuclein pathology in a human brain-chip to assess blood-brain barrier disruption. Nature communications, 2021. 12(1): p. 5907.
- Lee, S.W.L., et al., Modeling nanocarrier transport across a 3D in vitro human blood‐brain–barrier microvasculature. Advanced healthcare materials, 2020. 9(7): p. 1901486.
- Park, T.-E., et al., Hypoxia-enhanced Blood-Brain Barrier Chip recapitulates human barrier function and shuttling of drugs and antibodies. Nature Communications, 2019. 10(1): p. 2621.
- Bahmaee, H., et al., Design and Evaluation of an Osteogenesis-on-a-Chip Microfluidic Device Incorporating 3D Cell Culture. Frontiers in Bioengineering and Biotechnology, 2020. 8.
- Sun, S., et al., Modeling human HSV infection via a vascularized immune-competent skin-on-chip platform. Nature communications, 2022. 13(1): p. 5481.
- Kim, J.J., et al., A microscale, full-thickness, human skin on a chip assay simulating neutrophil responses to skin infection and antibiotic treatments. Lab on a Chip, 2019. 19(18): p. 3094-3103.
- Li, Z.A. and R.S. Tuan, Towards establishing human body-on-a-chip systems. Stem Cell Res Ther, 2022. 13(1): p. 431.
- Ronaldson-Bouchard, K., et al., A multi-organ chip with matured tissue niches linked by vascular flow. Nat Biomed Eng, 2022. 6(4): p. 351-371.
- Sequiera, G.L., et al., Development of iPSC-based clinical trial selection platform for patients with ultrarare diseases. Science Advances, 2022. 8(14): p. eabl4370.