Microfluidic pump flow profiles: a comparative review
Controlling microfluidic pump flow is essential for biological assays, chemical analysis, and material synthesis applications. Different pumping systems can generate various flow profiles, each with unique characteristics suited to specific experimental requirements.
Microfluidic pump flow can be regular or irregular. The flow pattern of a regular flow is steady or pulsatile, whereas the flow pattern of an irregular flow is disturbed or oscillatory. For steady flow, a certain shear stress (τ) is produced. For pulsatile and reciprocating flow, cyclic change in the shear stress level is maintained, with a higher level for pulsatile flow than for reciprocating flow (Figure 1) [1].

Microfluidic pump flow manipulation strategies can be categorized as passive and active methods. Passive manipulation methods mainly rely on microfluidic design, microchannel geometry, and internal forces to affect liquid flow without the need for external forces or triggers. Examples of passive manipulation are hydrostatic pressure and capillary-driven flow. On the other hand, active methods utilize an external force field to generate flow, such as syringe, peristaltic, pressure, gear, electroosmotic, and centrifugal pumps.
Here’s an overview of microfluidic flow profiles generated by passive and active manipulation.
Passive manipulation of microfluidic flow
Capillary-driven flow
Flow profile: Passive flow driven by capillary action
Working principle: Fluids move through microchannels due to capillary forces, without the need for external microfluidic pump or pressure. Surface tension and channel geometry control the flow.
Characteristics/Considerations:
- No external microfluidic pump or power source required.
- Simple, self-sustained flow.
- Ideal for portable, low-cost devices.
- Limited to slow and steady flow rates.
- Difficulty controlling or adjusting flow once initiated.
- Flow is influenced by channel geometry, liquid properties (viscosity, surface tension), and the material wetting properties.
Applications:
- Microfluidic paper-based analytical devices (μPADs), lateral flow assays (e.g., pregnancy tests), and low-cost point-of-care diagnostics [2, 3].
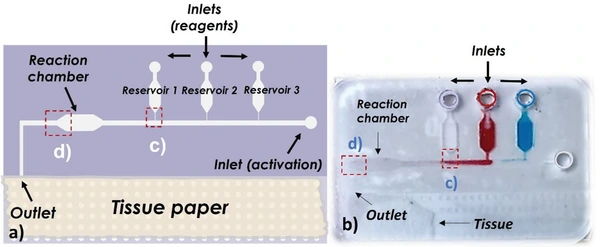
Hydrostatic pressure
Flow profile: Slow, laminar flow
Working principle: The hydrostatic pressure method is based on differences in fluid height to generate fluid flow through a microchannel.
Characteristics/Considerations:
- No external microfluidic pump or power source required.
- Passive flow is essential in applications where active components might introduce noise.
- Laminar flow suits applications requiring limited mixing.
- Can generate low flow rates only.
- Flow rate control is limited to fluid column height and liquid volume. Adjusting flow requires manual intervention.
- Flow decreases over time as the difference in height between the two reservoirs decreases.
- Flow is disrupted when adjusting reservoir height or refilling reservoirs.
- Flow rate can be controlled by a programmable rocking platform. In this case, the flow rate depends on the rocker speed and tilt angle.
Applications:
- Cell culture studies, disposable diagnostic devices, and diffusion-based experiments requiring a slow, steady, and laminar flow.
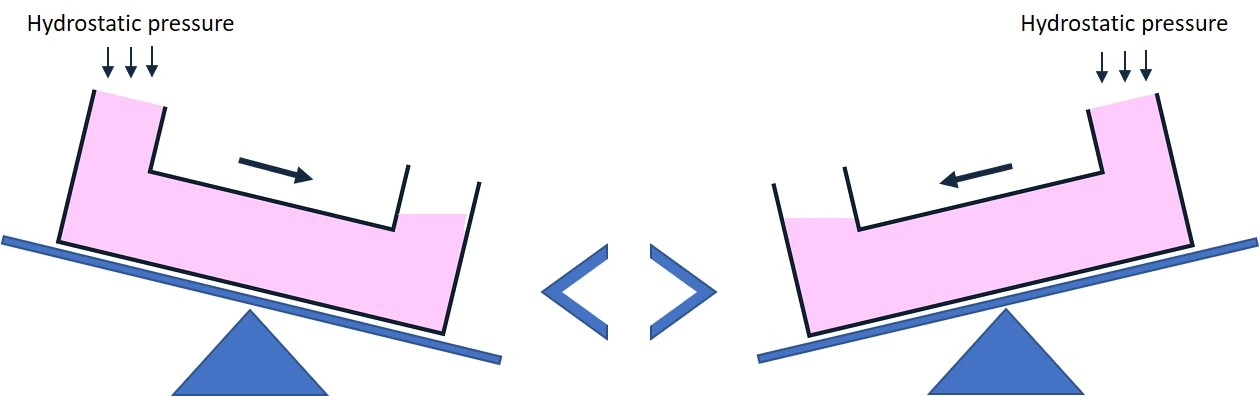
Active manipulation of microfluidic pump flow
Syringe microfluidic pump
Pump flow profile: Oscillating, pulsatile.
Working principle: Syringe pumps can both push-in (infusion) and pull-out (withdrawal) fluid through a microfluidic channel using an electric motor pushing a syringe plunger at a set rate. With programmable syringe pumps, users can go beyond simple infusion and withdrawal operations and create customized pumping methods with simple to complex flow profiles.
Characteristics/Considerations:
- The accuracy is 0.25% max with near pulseless syringe pumps
- The flow rate is constant over time, even with varying microfluidic resistance.
- The syringe pump is suitable for precise flow control and low flow rates (µL/min to mL/min).
- Experiment length is limited by the syringe volume unless an infusion-withdraw syringe pump is used.
- Flow is disrupted when the syringe is empty and during push/pull transition.
- The flow rate is not monitored in real-time; calibration may be required.
Applications:
- Chemical reactions, microfluidic cell assay, drug delivery studies, or analytical measurements where slow reagent addition is needed [5].

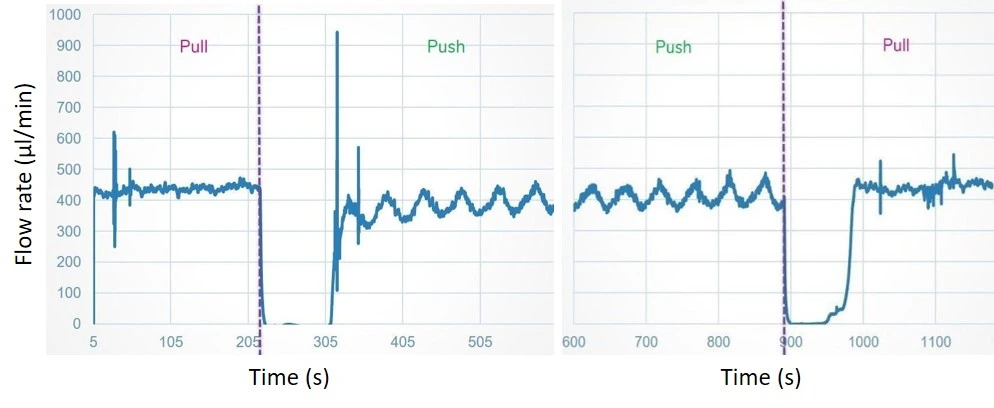
Peristaltic microfluidic pump
Pump flow profile: Pulsatile flow.
Working principle: Peristaltic pumps are versatile, efficient, and reliable. Fluid is pushed through a flexible tubing in the pump head by the compression and release of rollers in the peristaltic pump. Pressure is applied to the liquid within, causing it to be squeezed through the length of the tubing and controlling its speed.
Characteristics/Considerations:
- Flow is continuous.
- Can handle shear-sensitive fluids like biological samples.
- Fluid displaced volume is limited by the volume of the reservoirs in a non-recirculation setup.
- Continuous unidirectional fluid recirculation is possible with one reservoir as an inlet and outlet.
- The amplitude of pulsed or uneven flow may not suit all applications. However, this can be smoothed out with certain tubing configurations or additional dampeners.
- Flow control is not monitored in real-time and is less stable over time, requiring repeated flow rate calibration.
- Peristaltic pumps cannot reach low flow rates (less than 1 µL/min).
- Usually less accurate than syringe pumps and pressure controllers.
Applications:
- Ideal for applications that require continuous flow over long periods, such as perfusion in cell culture systems, or nutrient delivery.
- Ideal for handling chemicals, or hazardous materials, as the fluid remains isolated from the pump and is transported through disposable tubing [6].
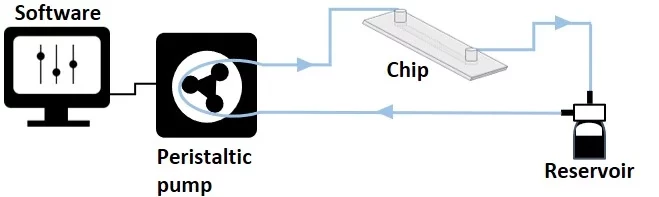
Pressure microfluidic pump
Pump flow profile: A variety of flow profiles is possible: pulsatile, steady, stepwise, customized.
Working principle: Pressure-driven pumps control the pressure applied to a fluid reservoir, driving fluid through microfluidic channels.
Characteristics/Considerations:
- High precision and fast response to flow control.
- Flow rate control can be achieved with a flow sensor feedback loop.
- Suitable for both low and high flow rates.
- Continuous operation as long as the pressure source is stable.
- Fluid displaced volume is limited by the volume of the reservoirs in a non-recirculation setup.
- Continuous fluid circulation allows infinite looping of liquid (volume is not consumed).
- Bidirectional fluid flow in a recirculation setup, can be made unidirectional with the use of a valve system (check our automated recirculation system).
- Flow rate is influenced by changes in channel resistance or viscosity; thus, adjustments may be needed.
Applications:
- Droplet generation, rapid mixing, and cell-based assays where pressure changes allow dynamic control over the flow.
- Unidirectional recirculation for cell perfusion using a rotary valve, 3/2 valves, or check valves [7].
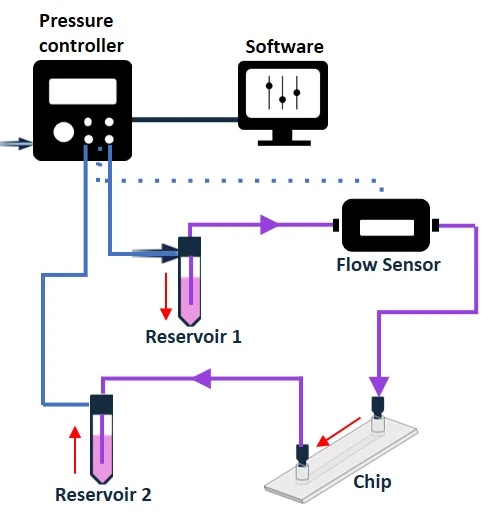
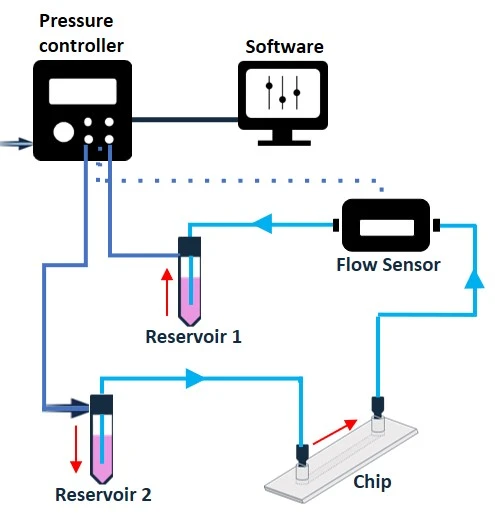
Figure 7: Recirculation setup with a pressure pump. The flow is bidirectional without the use of a valve system.
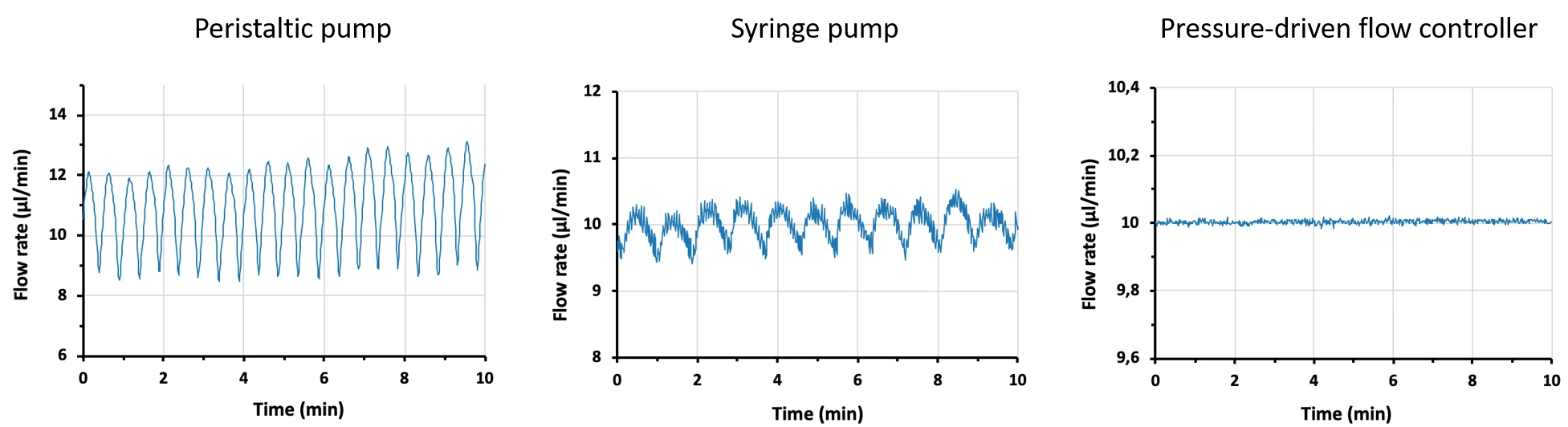
Figure 8: Comparison between flow profiles generated by a peristaltic pump, syringe pump, and pressure pump. Flow was measured by Galileo flow sensor.
Gear microfluidic pump
Pump flow profile: Steady, near-constant flow
Working principle: Microfluidic gear pumps consist of two or more gears that rotate against each other inside a tightly fitted housing. As the gears rotate, fluid gets trapped between the teeth of the gears and the housing, moving it from the pump inlet to the outlet.
Characteristics/Considerations:
- Smooth, low-pulsation flow.
- High precision and fast response to flow control.
- Steady flow even when the system encounters variations in backpressure or fluid resistance.
- Suitable for medium to high-viscosity fluids.
- The flow rate can be adjusted by changing the speed of the gears’ rotation.
- Not suitable for low-viscosity fluids.
- Limited flexibility in adjusting the volume of displaced fluid per revolution, which depends on the design of the gears.
Applications:
- Microreactors and for precise recirculation of drugs in medical devices.
- Microfluidic PCR (Polymerase Chain Reaction) and chromatography, that require low-pulsation, and constant flow [8].
An example of a gear pump is the micro annular pump developed by HNP Mikrosysteme. It consists of inner and outer gears assembled within the pump casing. When the inner gear is rotated, the outer gear is turned in the same direction. This pump’s plus is that the tight tolerances between the gears prevent liquid from leaking out on the discharge side, and the incremental gear rotation makes it easy to control the amount of displaced liquid.
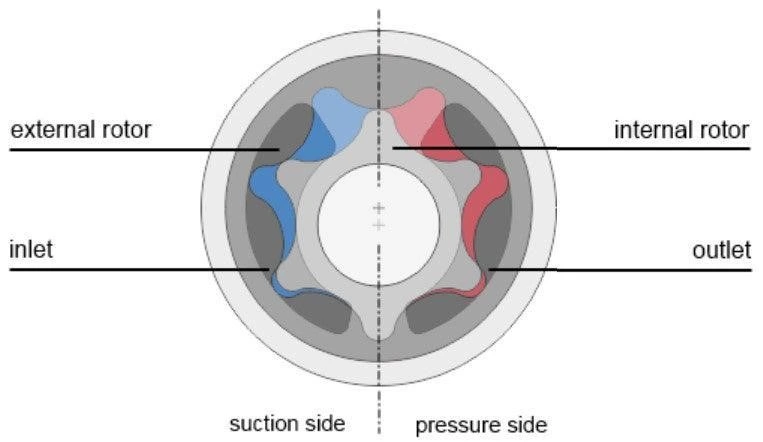
Figure 9: Principal components of a gear pump. (Image taken from Darwin Microfluidics website).
Electroosmotic microfluidic pump
Pump flow profile: Constant and pulseless flow, or tunable flow controlled by an electric field
Working principle: Electroosmotic pumps move fluids through microchannels by applying an electric field, generating flow due to the movement of ions.
Characteristics/Considerations:
- No moving parts, leading to minimal mechanical wear and tear.
- Fine control over very small (nL to pL) volumes.
- Sensitive to changes in fluid properties like pH, ionic strength, and channel surface conditions.
- Limited to polar and conductive fluids.
Applications:
- Single-cell genomics or proteomics, where small sample volumes must be precisely controlled and analyzed.
- Electrophoresis and capillary electrophoresis systems for separating biomolecules or ionic species based on size and charge.
- Affinity chromatography for protein or peptide separation and purification [9].
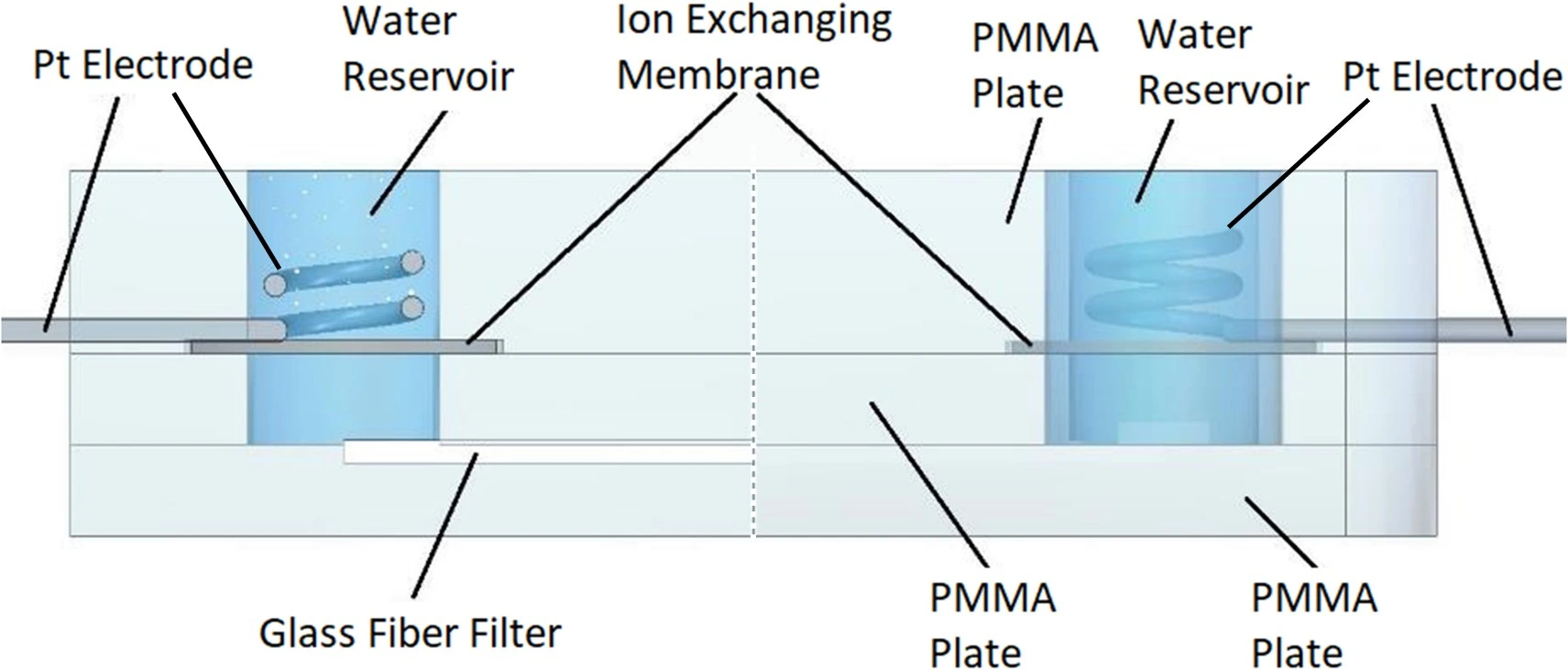
Centrifugal microfluidic pump
Pump flow profile: Centrifugal, steady, pulseless flow.
Working principle: Centrifugal pumps drive fluid flow using the rotational force applied to a microfluidic disk or platform. Fluids are pushed radially outward as the platform spins.
Characteristics/Considerations:
- Simple and can control multiple fluids in parallel.
- No external tubing or pumps required.
- Flow is continuous with the forward and backward fluid movement to the different compartments.
- Limited to applications on rotating platforms.
- Flow rate depends on rotational speed and platform design.
Applications:
- Point-of-care diagnostics, sample preparation, rapid mixing, and multiplexed assays in disk-based microfluidic platforms [11].
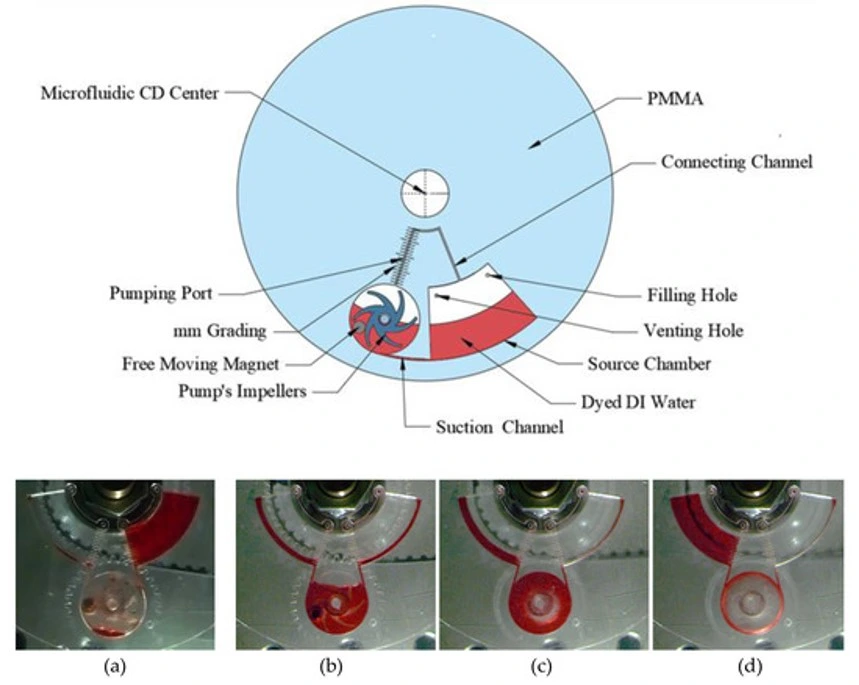
Other microfluidic pump mechanisms
Most microfluidic pumps are based on a piezoelectric mechanism or proportional valves.
Piezoelectric mechanism
A piezoelectric mechanism is based on the inverse piezoelectric effect, where an applied electric field causes a piezoelectric material to deform. This deformation drives a diaphragm within the pump, creating air or liquid compression that pushes the fluid through the pump chamber.
Characteristics:
- Very fine control over fluid volumes and flow rates by modulating the frequency and voltage of the applied electric signal.
- Fast response time due to the direct actuation mechanism.
- Often binary (fully open/close).
The MIC has 2 micropumps that function using pneumatic piezoelectric technology. Our Caterpillar pressure pump is based on a pneumatic piezoelectric micropump with air compression.
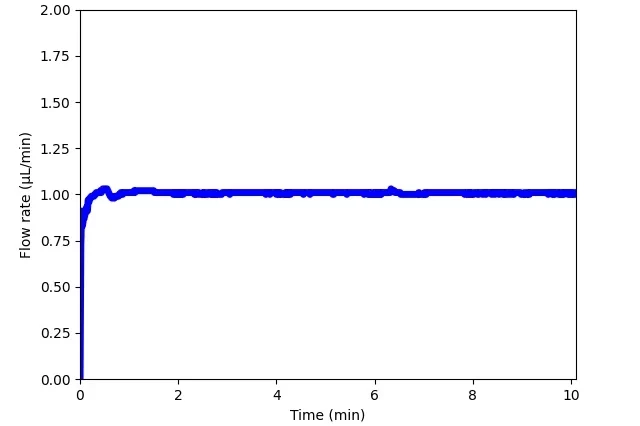
Our mini pump uses a pneumatic piezoelectric micropump with liquid compression.
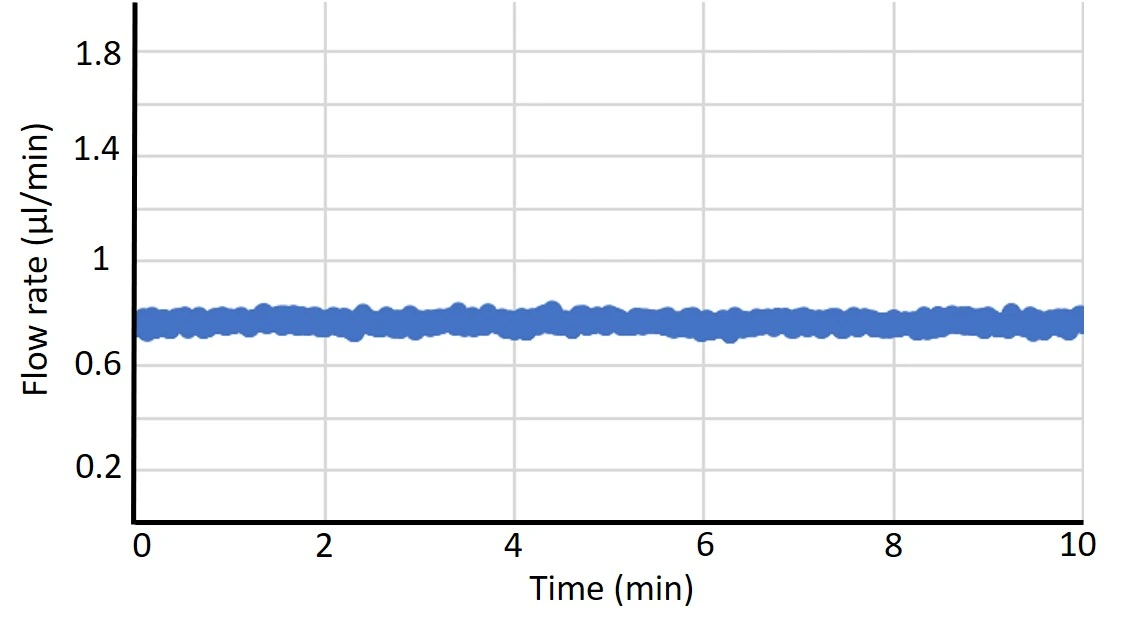
Proportional valves
Proportional valves operate based on electromagnetic principles. When current passes through a solenoid actuator, the actuator generates a magnetic field, which moves the valves and pulls them against the force of the return mechanism. The valve opening adjusts continuously, providing a proportional flow rate based on the input. Proportional valves allow for precise, continuous fluid flow control, unlike binary valves that are open or closed.
Characteristics:
- Provide a broad range of continuous flow rates
- Precision depends on the resolution of the proportional actuator, but they may not achieve the same fine granularity as piezoelectric valves in certain applications.
- Slower response times compared to piezoelectric valves because of the mechanical movement of components.
- Better for applications where steady flow adjustments are needed over time.
Our cell culture pressure pump is based on proportional valves.
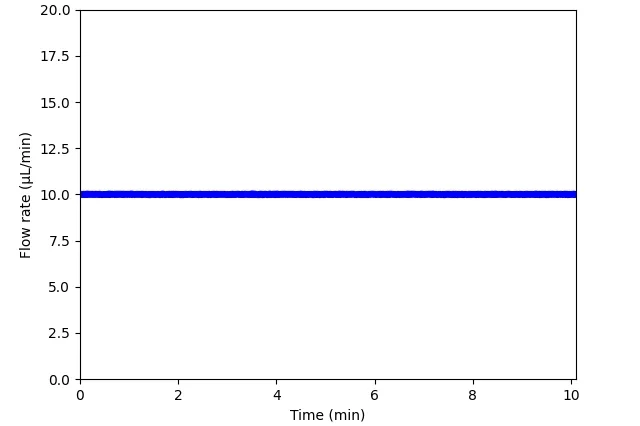
Some pumps are based on piezo-controlled proportional valves (eg. Hoerbiger) combining both mechanisms.
Microfluidic pump flow profile summary
Pump system |
Flow profile |
Flow rate |
Flow rate control |
Volume capacity |
Flow continuity |
Capillary-driven |
Passive |
Suitable for low flow rates |
Slow, self-driven by surface tension |
Not limited by volumes |
Continuous flow without interruptions |
Hydrostatic pressure |
Laminar |
Suitable for low flow rates |
None to limited; Can be controlled using a programmable rocking platform. |
Limited by reservoir height, requires refilling |
Disrupted when adjusting reservoir height or refilling reservoirs |
Syringe pump |
Oscillating, pulsatility depends on the pump |
Suitable for low flow rates |
Precise, controlled by the pump. |
Limited by syringe volume, requires refilling |
Disrupted when pull/push transition or when syringe empties |
Peristaltic pump |
Pulsatile, can be reduced with a dampener |
Not suitable for low flow rates |
Moderate, controlled by the pump and tubing selection. |
Limited by the volume of the reservoirs * |
Continuous flow without interruptions |
Pressure pump |
Highly stable profile; Variety of flows possible: pulsatile, steady, stepwise, custom |
Suitable for both low and high flow rates |
Very precise; Uses a flow sensor feedback loop |
Limited by the volume of the reservoirs ** |
Continuous flow, can be disrupted when refilling reservoirs |
Gear pump |
Smooth, near-constant |
Suitable for low flow rates |
Precise, controlled by the gears design |
Limited by the volume of the reservoirs |
Continuous flow without interruptions |
Electroosmotic pump |
Constant, pulseless |
Suitable for low flow rates |
Steady, controlled by an electric field |
Limited by the volume of the reservoirs |
Continuous flow, can be disrupted when refilling reservoirs |
Centrifugal pump |
Centrifugal, pulseless |
Suitable for high flow rates |
Radial, depends on rotational speed |
Not limited by volumes |
Continuous flow forward and backward without interruptions |
*Infinite volume looping in a recirculation setup with one reservoir
**Infinite volume looping in a recirculation setup with two reservoirs and an additional valve system for unidirectional flow
This review was written by Celeste Chidiac, PhD.
Published in December 2024.
Contact: Partnership[at]microfluidic.fr
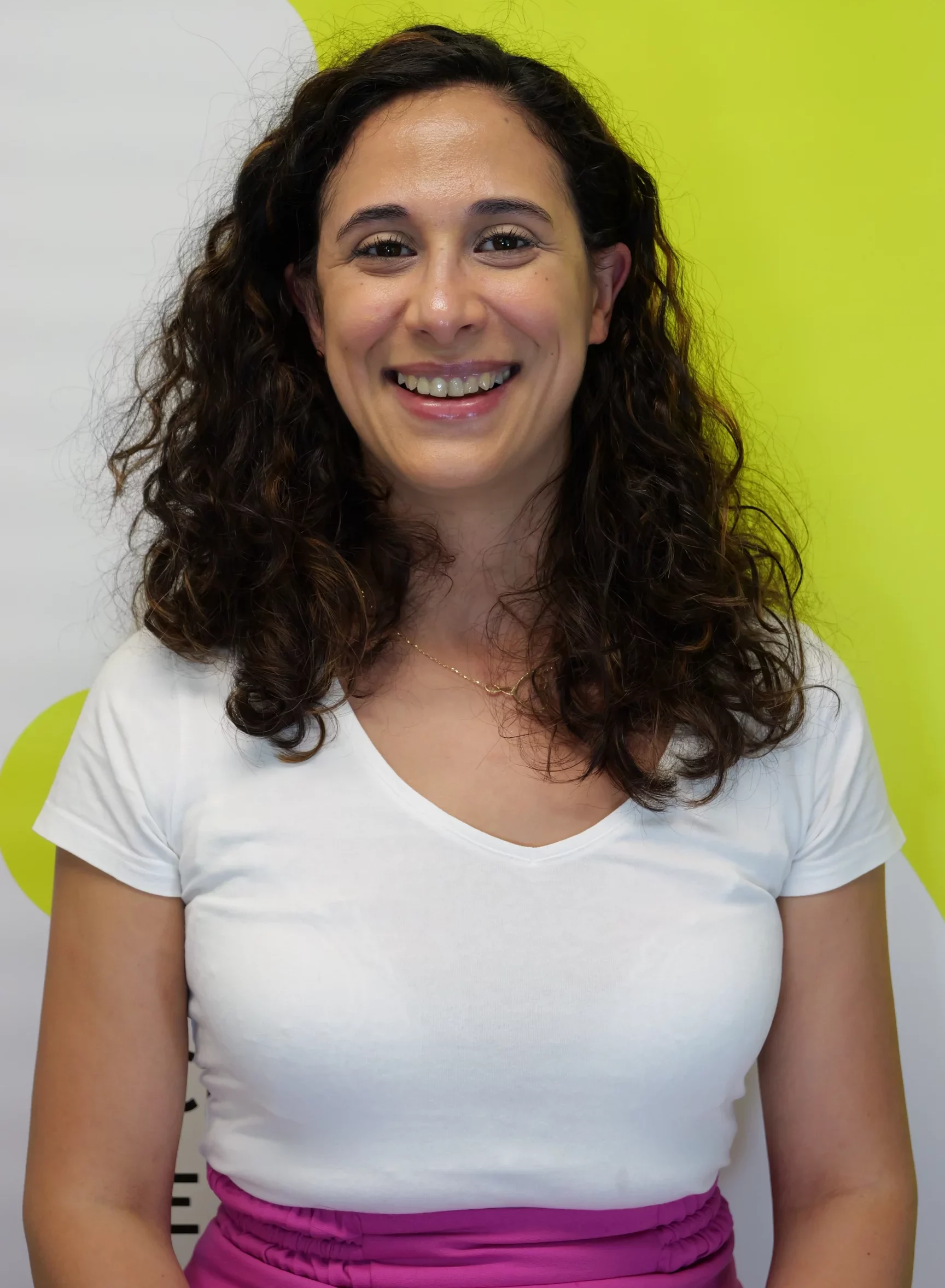
References
- Hsieh, H.-J., et al., Shear-induced endothelial mechanotransduction: the interplay between reactive oxygen species (ROS) and nitric oxide (NO) and the pathophysiological implications. Journal of Biomedical Science, 2014. 21(1): p. 3.
- Mérian, T., et al., Development and surface characterization of an electrowetting valve for capillary-driven microfluidics. Colloids and Surfaces A: Physicochemical and Engineering Aspects, 2012. 414: p. 251-258.
- Khamcharoen, W., et al., Capillary-driven microfluidic device integrating recombinase polymerase amplification for human papillomavirus detection. Sensors and Actuators B: Chemical, 2024. 401: p. 135016.
- Makhinia, A., et al., On-Demand Inkjet Printed Hydrophilic Coatings for Flow Control in 3D-Printed Microfluidic Devices Embedded with Organic Electrochemical Transistors. Advanced Materials Technologies, 2023. 8(15): p. 2300127.
- Haque, M.E., et al., Effects of syringe pump fluctuations on cell-free layer in hydrodynamic separation microfluidic devices. Physics of Fluids, 2021. 33(7).
- Behrens, M.R., et al., Open-source, 3D-printed Peristaltic Pumps for Small Volume Point-of-Care Liquid Handling. Scientific Reports, 2020. 10(1): p. 1543.
- Sullender, C.T., et al., Using pressure-driven flow systems to evaluate laser speckle contrast imaging. Journal of Biomedical Optics, 2022. 28.
- Alam, M.N.H.Z., et al., Design and fabrication of a 3D printed miniature pump for integrated microfluidic applications. International Journal of Precision Engineering and Manufacturing, 2017. 18: p. 1287-1296.
- Wang, X., et al., Electroosmotic pumps and their applications in microfluidic systems. Microfluid Nanofluidics, 2009. 6(2): p. 145.
- Ecker, R., et al., Microfluidic High-Power Electroosmotic Pumps Based on Glass Fiber Filters. IEEE Sensors Letters, 2024. 8(9): p. 1-4.
- Al-Halhouli, A.a., et al., Development of Active Centrifugal Pump for Microfluidic CD Platforms. Micromachines, 2020. 11(2): p. 140.