Microfluidic tumor-on-chip systems for breast cancer research
Breast cancer is one of the most frequent malignant tumors among women worldwide.
In the early stage of breast cancer, neoplastic epithelial cells accumulate in the lumen of the mammary duct, where they form pre-invasive cancerous lesions known as ductal carcinoma in situ (DCIS).
Ductal means that the cancer starts inside the milk ducts. Progression of invasive breast cancer occurs when the tumor cells in DICS penetrate the basement membrane and invade the surrounding tissue [1].
Introduction to microfluidic breast tumor-on-chip systems for breast cancer studies
The transition from in situ ductal carcinoma to invasive ductal carcinoma brings aberrant changes in matrix remodeling, paracrine signaling, and immune responses [2-4].
Metastatic breast cancer occurs when the cancer spreads from the breast to another part of the body, such as the lungs, liver, bone, etc.
The tumor microenvironment comprises a dynamic network of extracellular matrix (ECM) proteins associated with fibroblast cells, bone marrow-derived cells, endothelial cells, and infiltrating immune cells [5].
These stromal cells remodel the ECM and provide mechanical and chemical cues to the tumor cells. Despite extensive research and enormous efforts in drug discovery over the last few decades, symptoms and treatment of this stage of breast cancer are difficult.
This is partly due to the need for a better understanding of the tumor microenvironment and the high cost of developing a new anti-cancer drug [6,7]. Circulating tumor cells can likewise be studied using microfluidic approaches.
Cancer research typically depends on 2D in vitro studies and animal models to investigate the tumor mechanism, angiogenesis, invasion, and metastasis [8]. However, two- and three-dimensional in vitro models have been widely used for screening anti-cancer drugs and studying cell signaling, proliferation, migration, and drug responses, including altered protein/gene expression [9].
These in-vitro models may not be able to mimic the exact tumor microenvironment and are thus not entirely suitable for studying the effect of complex spatial organization and cell interaction. However, these 3D tumor models create tumor microstructures and can capture cell-cell and cell-matrix interactions.
Although 3D models benefit from recapitulating the tumor cell organization, they still lack the vasculature and fluid shear stress found in vivo [10]. On the other hand, animal models can provide essential in vivo information on tumor growth and response to drug molecules but are very costly, and results may vary between animals. In addition to the ethics surrounding in vivo model usage, serious concerns still exist over their biological relevance to humans [11].
To significantly improve cancer therapy, more effective approaches to screening anti-cancer drug candidates and a better understanding of the tumor microenvironment using advanced technologies, including microfluidics and organ-on-chip technology, are necessary.
Microfluidic tumor-on-a-chip systems
A tumor-on-chip is a microfluidic-based 3D system capable of recapitulating tumor cells’ biological activities, mechanical properties, and physiological responses [12].
Unlike conventional 3D in vitro models, tumor-on-a-chip utilizes fluid dynamics to enhance long-term culturing capabilities under physiological conditions.
Typically, tumor-on-chip incorporates a 3D culturing system with fluid tubing and channels to control the delivery of nutrients and remove waste from the system to mimic the microenvironments of tumor cells grown in vivo.
Breast cancer tumor-on-chip devices
To mimic the 3D structural organization of the human mammary duct, Choi et al. (2015) designed a microdevice consisting of an upper and a lower microchannel separated by a semi-permeable membrane that mimics the basement membrane.
This device used the compartmentalized 3D microfluidic device for drug screening on cocultured breast tumor spheroids with human mammary duct epithelial cells and mammary fibroblast cells. In this design, the upper microchannel represented the ductal lumen, which allows a continuous flow of culture media that is required for growing and maintaining the mammary epithelial cells and DCIS spheroids on the upper side of the Extracellular Matrix (ECM) membrane (Fig 1).
A stromal layer impregnated with mammary fibroblast is formed on the lower side of the ECM membrane and perfused with culture media through the lower microchannel to mimic the vascular compartment of capillaries in the mammary stroma.
A collagen membrane separates the upper and lower microchannels. In this microfabricated device, the cocultured cells were maintained by a continuous flow of culture media at the physiological rate of interstitial flow (2-70 µl/hr).
They also studied the impact of the clinical chemotherapeutic anticancer drug Paclitaxel related to the size of Ductal Carcinoma in situ (DCIS). They showed that Paclitaxel can arrest tumor cell proliferation and inhibit the growth of DCIS in the tumor microenvironment [1].
This micro-engineered disease model successfully represented the complexity of breast cancer pathophysiology and could be used as a tool to systematically study the invasiveness of DCIS in a breast cancer microenvironment similar to that found in the patient’s body.
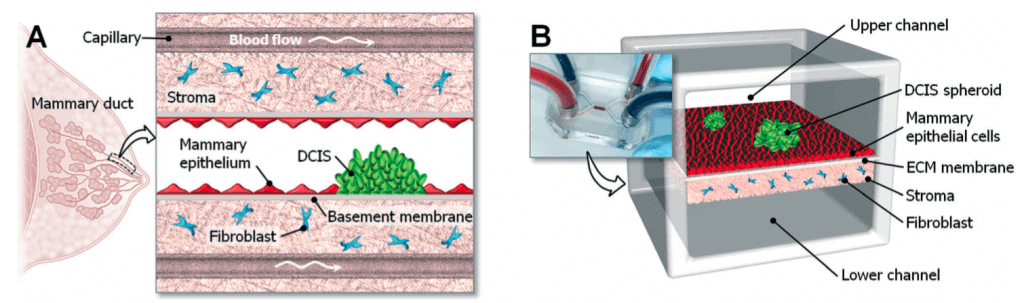
Paracrine signaling and extracellular matrix activation in breast cancer research
Similar to the above-described approach, Gioiella et al. (2016) also used a microfluidic platform to simulate breast cancer on a chip and to replicate epithelial-stroma interactions (cell phenotype, extracellular matrix composition, and organization, macromolecular transport) that occur in the healthy stroma during the invasion of malignant epithelial breast cells. In this device, stroma and epithelial tumor tissue were separated by an interface that allows physical contact.
This device used normal and cancer-activated fibroblast cells to produce cancer microtissues. When these cells are cocultured, normal fibroblast cells differentiate into myofibroblasts after physical contact with cancer cells in the microfluidic device. The group likewise investigated the expression of Matrix Metalloproteinase (MMPs) during cancer invasion.
The paracrine signaling among cancer cells and fibroblast cells induced the production of MMPs (MMP2 and MMP9) that activated the migration of cancer cells in the 3D environment. These MMPs can degrade collagen IV and weaken the basement membrane [13].
The degradation products of the extracellular matrix, including collagen IV fragments, provide signaling cues to regulate the tumor cell motility. The effects of different ECM components (collagen, hyaluronic acid (HA), and fibronectin) on tumor interactions were likewise evaluated in the tumor microenvironment.
A significant number of studies report that deposition of HA and fibronectin increases in various types of cancer tissues, including breast cancer tissues [14]. The rate of HA synthesis is much higher in cancer tissue compared to normal tissue. Increased HA levels in cancerous tissue promote cancer cell invasion, migration, metastasis, and tumor-stroma interactions [13]. HA levels increase from in situ ductal carcinoma to later stages of invasive cancer sites.
Co-culture systems for breast cancer migratory analysis
Mi et al. (2016) have presented a novel microfluidic system where they establish an in vitro co-culture model of breast cancer cells and human mammary epithelial cells (HMEpiC) that mimics different regions of a metastatic breast tumor (Fig 2).
This breast tumor model was used to study the effects of the anti-cancer drugs Paclitaxel and Tamoxifen on tumor migration. Drug screening using microfluidics is an innovative and promising approach, and the results showed that migration of cancer cells (MDA-MB-231) was increased after coculture with human mammary epithelial cells.
The secretion of proinflammatory cytokines increased when breast cancer cells were cocultured with breast epithelial cells, which altered their cell morphology and modulated cell migration.
The co-culture also decreased the secretion of Cytokeratin-14 (a characteristic protein of epithelial cells) and morphological changes in HMEpiC cells [15]. The significant inhibition of migration of the cancer cells was observed after adding different concentrations of Paclitaxel and Tamoxifen.
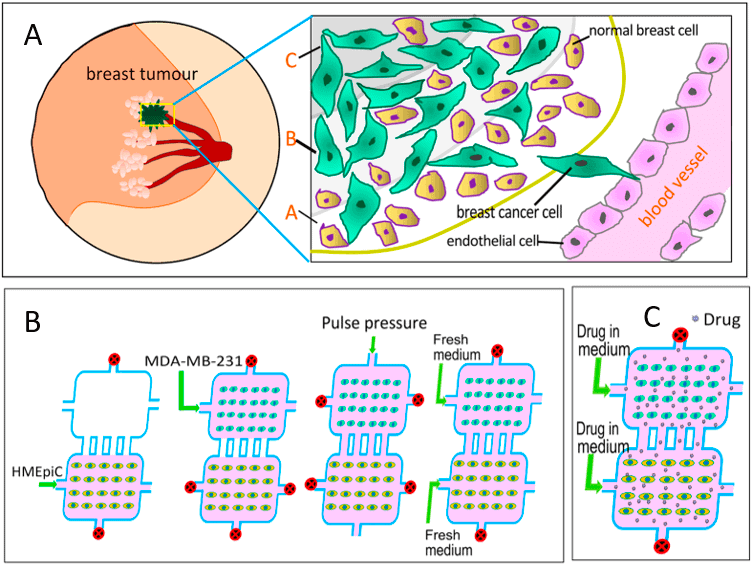
Future perspectives of breast-tumor-on-chip systems
Ultimately, the key advantage of breast cancer-on-chip is to grow artificial breast tissues on-chip. Many therapeutic approaches can be tested faster and cheaper than animal models or human subjects.
Tumor-on-chip technology also holds the key to reducing the use of animals in pharmacodynamic and pharmacokinetic investigations, as well as toxicology studies.
The devices will allow the design and testing of more preventive interventions that may lead to breakthrough advances in breast cancer prevention and treatment.
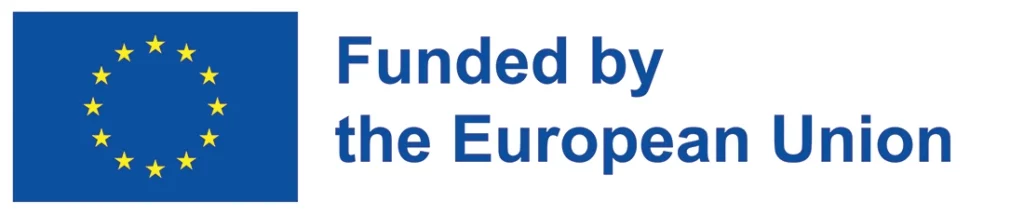
Review written thanks to the support of the MTOAC H2020-MSCA-IF-2017 – Marie-Curie Action:
« Individual Fellowships »
Grant agreement number: 795754
Written by Subia Bano, Postdoc
Contact:
Partnership[at]microfluidic.fr
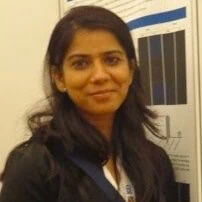
References
- Natalie Angier, “Physiologie. Le foie, cet organe à tout faire”, Courrier international (The New York Times), 13 juillet 2017
- Navarro VJ, Senior JR. Drug-related hepatotoxicity. N Engl J Med. 2006;354:731–739.
- Hepatitis B Fact sheet N°204. who.int. July 2014. Retrieved 4 November 2014
- Toxicol Res (Camb). 2013 Jan 1;2(1):23-39. Epub 2012 Nov 23. In vitro models for liver toxicity testing. Soldatow VY, Lecluyse EL, Griffith LG, Rusyn I.
- “the cure – liver on a chip”, link
- Weng YS, Chang SF, Shih MC, Tseng SH, Lai CH, 2017 Jul 21. Scaffold-Free Liver-On-A-Chip with Multiscale Organotypic Cultures.
- M. Trauner, J. L. Boyer, Physiol. Rev. 2003, 83, 633
- A. Esteller. World J. Gastroenterol. 2008, 14, 5641
- E. M. Huisman, T. V. Dillen, P. R. Onck, E. V. Giessen, Phys. Rev. Lett. 2007, 99, 208,103
- L. Lanzetti, Curr. Opin. Cell Biol. 2007, 19, 453.
- J. D. Humphrey, E. R. Dufresne, M. A. Schwartz, Nat. Rev. Mol. Cell Biol. 2015, 15, 802.
- E. L. LeCluyse, R. P. Witek, M. E. Andersen, M. J. Powers, Crit. Rev. Toxicol. 2012, 42, 501.
- Rinella ME, June 2015, Nonalcoholic fatty liver disease: a systematic review.
- Manuele Gori, Maria Chiara Simonelli, Sara Maria Giannitelli, Luca Businaro, Marcella Trombetta, and Alberto Rainer, 2016, Investigating Nonalcoholic Fatty Liver Disease in a Liver-on-a-Chip Microfluidic Device
- A. S. Khazali, A. M. Clark, A. Wells, June 2017, A Pathway to Personalizing Therapy for Metastases Using Liver-on-a-Chip Platforms
- Written by Emilie Grandfils, corrected by Julie Cavallasca, under the supervision of Dr. Guilhem Velve Casquillas