Actuation concepts for in vivo-like mechanical strain
Published on May 26th 2020
Abstract
Various actuation concepts can be used to generate membrane stretching. Mimicking stretching forces in vitro for cell culture is often required, for example, in lung-on-a-chip tissue culture (breathing), in heart-on-a-chip models (heartbeat), or gut-on-a-chip (peristaltic intestine movement).
We will briefly outline the most common and promising principles, including pneumatic, electromagnetic, piezoelectric, and dielectrophoretic actuation used in microfluidic organ-on-a-chip technology.
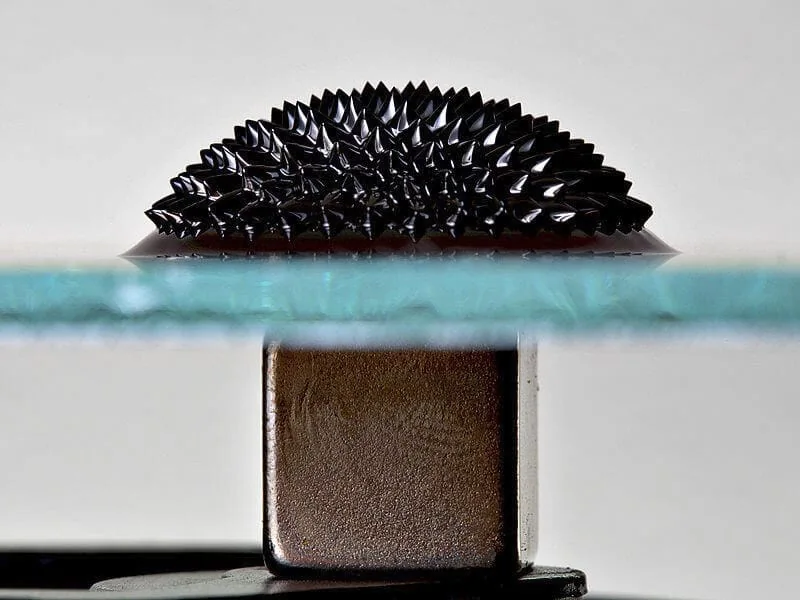
Introduction to actuation concepts for in vivo-like mechanical strain
Cells and tissues in the human body are naturally exposed to different mechanical forces. The forces range over multiple length scales. For instance, our bones and cartilage are exposed to compressive loads as we walk and move, and our blood vessels are continuously exposed to shear stresses due to vascular flow to cyclic strain due to blood pressure or lung tissue under mechanical strain during breathing. Mechanical forces are critical in cell function and regulation under normal and pathological conditions.
Cells sense and respond to mechanical forces through a process known as mechanotransduction. Mechanotransduction pathways typically involve complex biological signal cascades to convert mechanical cues into biochemical signals. The extracellular matrix (ECM) transmits many automated signals to the cells. The ECM is a complex network of proteins, glycosaminoglycans, and proteoglycans in which the cells reside. The major component of the ECM is collagen, but the composition varies depending on tissue type. The cells can sense mechanical forces through focal adhesions that couple the cells’ cytoskeletons to the ECM [1].
Although our understanding of mechanotransduction on a cellular and molecular level is limited, we know that mechanotransduction influences essential cellular functions such as growth, gene induction, protein synthesis, and cell death [2]. Abnormal forces or dysfunction in mechanotransduction contribute to developing several common diseases, including fibrosis, osteoporosis, hypertension, asthma, and cancer [3]. A better understanding of the underlying dynamics of tissue and disease progress can be critical in the search for new treatments and diagnostics.
In traditional in vitro cell culture models for disease modeling or drug screening, the role of mechanical forces is often overlooked. This is partly due to the practical challenges of delivering mechanical forces to in vitro cell cultures in a controlled manner. Recent advances in microtechnology, microfluidics, and biomaterials have enabled the development of new tools for exposing cells to mechanical inputs, such as shear stress, compression, and substrate strain, while monitoring cellular responses.
While several mechanical forces can be delivered to cells with organ-on-a-chip technology, this review will focus on how in vivo-like mechanical tensile strain can be produced in vitro. We summarize recent efforts to develop sophisticated cell stretching platforms using different actuation concepts and elaborate upon their practicality for organ-on-a-chip research.
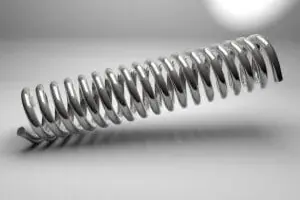
How actuation techniques can reproduce in-vivo mechanical strain
Traditional laboratory techniques to induce static or cyclic strain in cells include micropipettes, optical and magnetic tweezers, atomic force microscopes (AFM), or bendable micro pillars [4]. Following advances in soft lithography and state-of-the-art microtechnology, membrane-based stretching platforms quickly gained popularity. Such platforms contain a porous or non-porous elastic membrane that can be stretched and controlled to induce substrate strain. Membranes are standard components in microfluidic systems for actuation or valving – for instance, check valves.
Exploiting the know-how of membrane fabrication and integration into microfluidic systems, several groups have presented sophisticated membrane-based cell stretching platforms, producing either unidirectional [5], bi-directional [6], or three-dimensional [7] strain profiles.
The magnitude of mechanical strain in vivo varies mainly depending on many factors, including tissue type, age, and surrounding ECM. For in vitro experiments, desired strain magnitudes are typically in the range of 0.1-10% of linear elongation, as higher strain magnitudes lead to cell death.
As discussed previously by Guenat et al. (2018) [8], cross-comparison between different models can be difficult as strain can be quantified and presented in several ways: linear strain, surface deformation, or in-strain components (circumferential or radial). Assuming isotropic deformation, the formula below can be used to correlate uni-axial strain (or linear elongation) to bi-axial strain (surface expansion).
ε(SA )=(εLIN+1)^2-1
where〖ε(SA )=(SAf-SA0)/SA0, and εLIN=(Lf-L0)/L0, L0, and Lf are the lengths before and after elongation, respectively, and SA0 and SAf are the surface area before and after expansion [9].
Examples of actuation concepts
Various actuation concepts can be used to generate membrane stretching. We will briefly outline the most common and promising principles, including pneumatic, electromagnetic, piezoelectric, and dielectrophoretic actuation.
Other actuation forms, such as optical [10], electrothermal [11], and motor-driven [12], have also been reported for cell stretching. Several literature reviews have evaluated different actuation concepts [13, 14].
Pneumatic actuation
Pneumatic actuation concepts are widely used in microfluidics, as they can be fabricated with well-known microfabrication techniques and require only readily available equipment—an external positive or negative pressure source.
Many pneumatically actuated systems are based on the deformation of a thin membrane, often in polydimethylsiloxane (PDMS), that is deflected due to applied pressure. An example is the renowned organ-on-a-chip model by Huh et al. (2010), which is based on this actuation principle (illustrated in Figure 1A) [15].
An advantage of this method is that it can produce a homogenous strain profile across the membrane area. Shimizu et al. (2011) presented another sophisticated use of the pressure drop in a microchannel to generate a range of strain magnitudes for cell stretching within a device [16].
Piezoelectric actuation
Piezoelectric actuators present a high precision alternative to traditional stepper motors and they can produce broad ranges of computer controllable strains of high dynamics. Kamotani et al. (2008) integrated an array of cell stretching chambers where a flexible PDMS membrane in each micro chamber was deflected through piezoelectrically actuated pins [17].
The cell cultures in the micro chambers were in this way exposed to radial strain (Figure 1B). Actuation systems based on piezoelectric principle require adapted architectures to avoid direct contact between the system and the cell culture.
Electromagnetic actuation
The non-invasive nature of magnetic fields is an exciting concept in microfluidics. Electromagnetic force-driven actuators can offer controllable and programmable applications of strain but inherently induce a heating effect that must be managed. Kamble et al. (2017) developed a stretching platform consisting of a PDMS membrane with two embedded permanent magnets along the actuation axis, with the north poles facing each other to induce a repulsive force resulting in a static strain on the membrane [18]. They used two axially aligned electromagnets to actuate the permanent magnets to cause cyclic strain simultaneously. (Figure 1C).
Dielectric actuation
Poulin et al. (2016) demonstrated dielectric elastomer actuators for uniaxial stretching of cells [19]. The device comprised an elastomer membrane with cells atop, between two stretchable electrodes (Figure 1D).
An electrostatic force was generated as a voltage was applied between the two electrodes. This resulted in compression of membrane thickness and expansion of membrane area. This actuation method exposes the cells to an electrical force.
The study found no significant effect or damage of the fringing electric field on cell morphology. In a follow-up study (2018), the same group demonstrated how the dielectric actuation system could achieve highly high strain rates (870s-1) and be combined with live cell imaging to study fast dynamics [20].
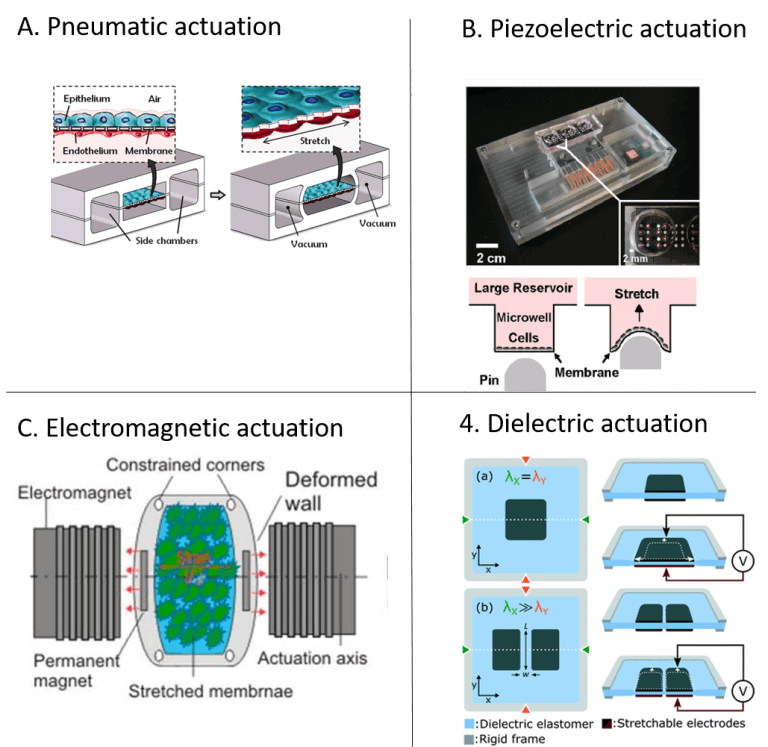
Conclusion and Perspectives
This review presented different actuation strategies to recreate in vivo-like tensile strain in vitro, focusing on integration in organ-on-a-chip technology. An important aspect related to the choice of actuation system is the compatibility with live imaging techniques to capture dynamic cellular processes. Data analysis in biology often relies partly or principally on fluorescent imaging. As cellular responses to mechanical stimuli can occur through highly dynamic processes, live-cell imaging in situ is critical for many mechanobiology studies [14].
Review done thanks to the support of the MaMi H2020-MSCA-ITN-2017-Action”Innovative Training Networks”, Grant agreement number: 766007
Author: Emma Thomée, PhD
Contact:
Partnership[@]microfluidic.fr
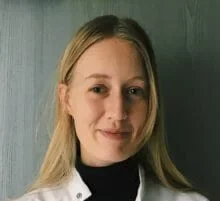
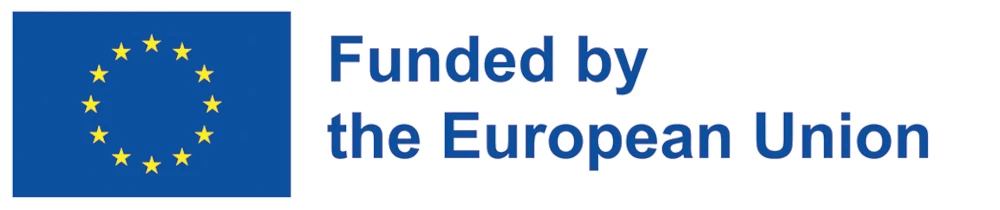
References
- K. A. Jansen, D. M. Donato, H. E. Balcioglu, T. Schmidt, E. H. J. Danen, and G. H. Koenderink, “A guide to mechanobiology: Where biology and physics meet,” BBA – Mol. Cell Res., vol. 1853, no. 11, pp. 3043–3052, 2015.
- D. E. Ingber, “Cellular mechanotransduction: putting all the pieces together again,” pp. 811–827, 2020.
- G. Y. H. Lee and C. T. Lim, “Biomechanics approaches to studying human diseases,” Trends Biotechnol., vol. 25, no. 3, pp. 111–118, Mar. 2007.
- D. Mohammed et al., “Innovative Tools for Mechanobiology: Unraveling Outside-In and Inside-Out Mechanotransduction,” vol. 7, no. July, 2019.
- C. H. E. N. R. E. I. W. An, S. E. O. K. C. Hung, and R. O. D. K. Amm, “Differentiation of Embryonic Stem Cells into Cardiomyocytes in a Compliant Microfluidic System,” vol. 39, no. 6, pp. 1840–1847, 2011.
- G. A. Giridharan et al., “Microfluidic Cardiac Cell Culture Model (μCCCM),” Anal. Chem., vol. 82, no. 18, pp. 7581–7587, Sep. 2010.
- C. Moraes, M. Likhitpanichkul, C. J. Lam, B. M. Beca, Y. Sun, and C. A. Simmons, “Microdevice array-based identification of distinct mechanobiological response profiles in layer-specific valve interstitial cells,” Integr. Biol., vol. 5, no. 4, pp. 673–680, Feb. 2013.
- O. T. Guenat, “Incorporating mechanical strain in organs-on-a-chip: Lung and skin,” vol. 042207, 2018.
- E. Roan and C. M. Waters, “What do we know about mechanical strain in lung alveoli?,” vol. 0001, pp. 625–635, 2020.
- I. Sraj et al., “Cell deformation cytometry using diode-bar optical stretchers,” J. Biomed. Opt., vol. 15, no. 4, pp. 1–7, Jul. 2010.
- G. Christopher, J. Yoo, N. Dagalakis, S. Hudson, and K. Migler, “Development of a MEMS based dynamic rheometer,” Lab Chip, vol. 10, pp. 2749–2757, Oct. 2010.
- L. Huang, P. S. Mathieu, and B. P. Helmke, “A stretching device for high-resolution live-cell imaging,” Ann. Biomed. Eng., vol. 38, no. 5, pp. 1728–1740, May 2010.
- H. Kamble, M. Barton, M. Jun, S. Park, and N.-T. Nguyen, “Cell stretching devices as research tools: Engineering and biological considerations,” Lab Chip, vol. 16, Jul. 2016.
- K. Kaarj, “Methods of Delivering Mechanical Stimuli to Organ-on-a-Chip,” 2019.
- D. Huh, B. D. Matthews, A. Mammoto, M. Montoya-Zavala, H. Y. Hsin, and D. E. Ingber, “Reconstituting Organ-Level Lung Functions on a Chip,” Science (80-. )., vol. 328, no. 5986, p. 1662 LP-1668, Jun. 2010.
- K. Shimizu, A. Shunori, K. Morimoto, M. Hashida, and S. Konishi, “Sensors and Actuators B: Chemical Development of a biochip with serially connected pneumatic balloons for cell-stretching culture,” Sensors Actuators B. Chem., vol. 156, no. 1, pp. 486–493, 2011.
- Y. Kamotani et al., “Individually programmable cell stretching microwell arrays actuated by a Braille display,” Biomaterials, vol. 29, no. 17, pp. 2646–2655, 2008.
- H. Kamble et al., “An Electromagnetically Actuated Double-Sided Cell-Stretching Device for Mechanobiology Research,” pp. 1–15.
- A. Poulin, C. Saygili Demir, S. Rosset, T. V Petrova, and H. Shea, “Dielectric elastomer actuator for mechanical loading of 2D cell cultures,” Lab Chip, vol. 16, no. 19, pp. 3788–3794, 2016.
- A. Poulin et al., “An ultra-fast mechanically active cell culture substrate,” no. April, pp. 1–10, 2018.