Multivesicular microfluidic vesicles for drug delivery and artificial cells
Published on 01 April 2022
Multivesicular vesicles (MVVs) are non-concentrically arranged vesicles-in-vesicle systems that can be prepared from bilayer-forming amphiphiles.
Although spontaneous formation of MVVs is possible, these hierarchical structures are usually obtained intentionally by guided molecular assembly in various well-known protocols.
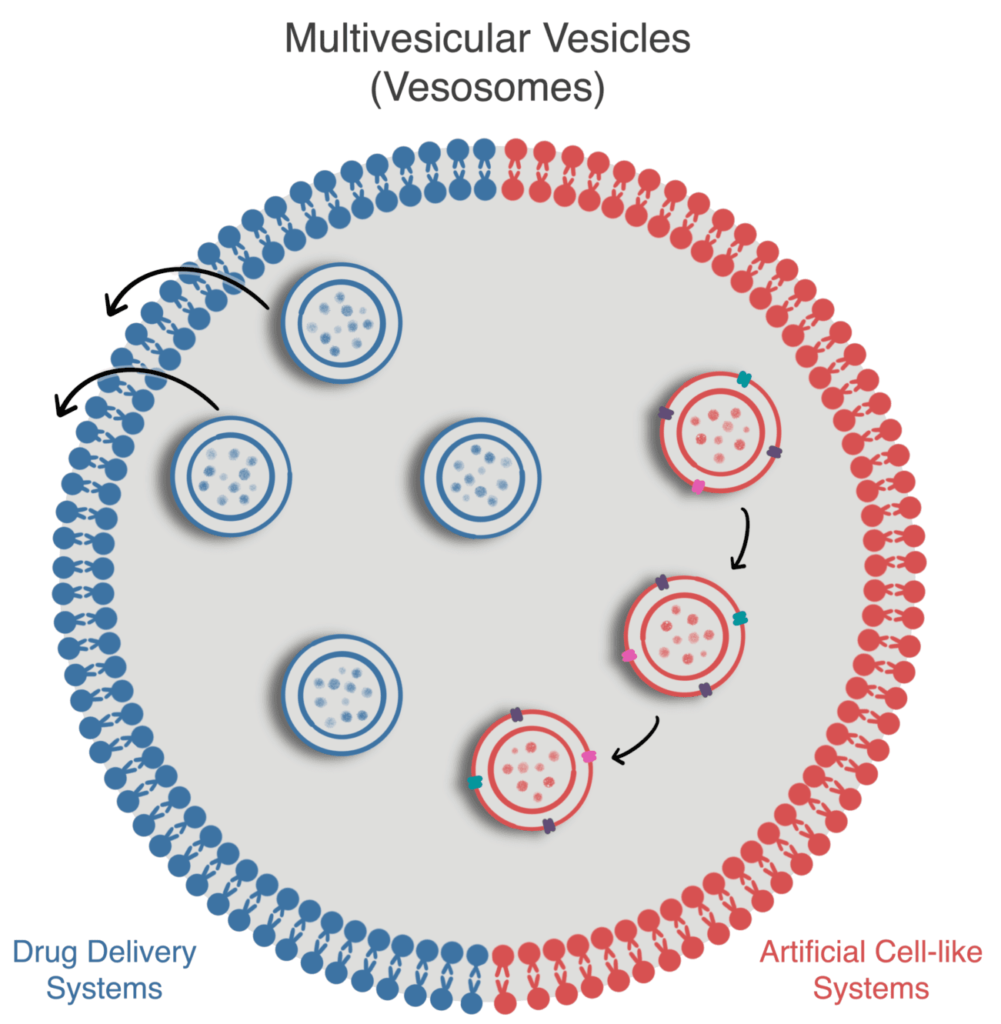
This review discusses the microfluidic method to generate MVVs and current applications as artificial cell-like systems or for drug delivery.
This review is based on the article entitled “Multivesicular Vesicles: Preparation and Applications,” written by Camila Betterelli Giuliano, Nemanja Cvjetan, Dr. Jessica Ayache, Prof. Dr. Peter Walde and published in the journal “ChemSystemsChem.” This work was supported by the Horizon 2020 Marie Skłodowska-Curie Actions Innovative Training Network (ITN) GA n° 813873, ProtoMet.
Introduction to multivesicular microfluidic vesicles
Liposomes are membrane compartments made with amphipathic molecules, often phospholipids, forming a bilayer [1, 2]. These compartments can be classified depending on their membrane structure and size (Fig.1).
For example, if they have only one bilayer, they are called unilamellar; if they have several, they are called oligolamellar or multilamellar blisters depending on the number of layers.
There is a greater interest in producing unilamellar vesicles due to their resemblance to the cell membrane and easier-to-predict membrane transport behavior [3]. They will be the focus of this review.
Unilamellar vesicles with sizes ranging from 30 to 50 nm in diameter are called “small or sonicated unilamellar vesicles (SUVs)”; from 50 to 500 nm, “large unilamellar vesicles (LUVs)”; and above 500 nm, “giant unilamellar vesicles (GUVs).”
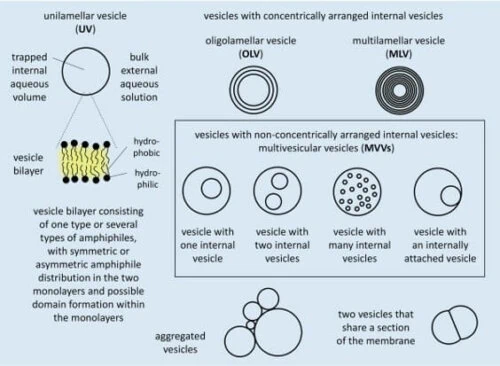
Unilamellar vesicles have been widely used in research as models for cell membrane studies and even reached the market as drug delivery systems [4].
Due to their versatility and well-established knowledge base, there has been an increased interest in studying more complex liposome-based systems.
Multivesicular microfluidic vesicles are non-concentrically arranged hierarchical compartments composed of smaller liposomes (SUVs or LUVs) inside more giant liposomes (LUVs or GUVs), which can also be called multivesicular vesicles (MVVs, Fig. 1).
These complex compartments are particularly interesting because, on one hand, they resemble more closely the hierarchical structure of cells [5] and, on the other hand, they allow for modulation of the release profile in drug delivery systems [6].
Multivesicular microfluidic vesicles production method
Many ways of obtaining multivesicular vesicles are extensively reviewed in Giuliano et al., 2020. Here, we will focus on microfluidic-based production due to the increased control and, consequently, reproducibility provided by the technology. These characteristics are translated and reinforced by the highly monodisperse populations of droplets and the high encapsulation efficiency allowed by microfluidics [7].
The underlying principle of producing liposomes in microfluidics is using water-in-oil-in-water droplets, or double emulsions, as templates [8].
Double emulsions are usually produced in glass capillaries [9] or in two-junction PDMS chips that are surface-treated to be part hydrophobic and part hydrophilic [8]. In the latter, the solution inside the water-in-oil-in-water droplet is pinched in the first junction by the oil phase.
The water-in-oil droplet travels in a hydrophobic channel until the second junction, where the oil phase will be lifted by the outer aqueous solution, forming the water-in-oil-in-water droplet (Fig. 2). The lipids are dissolved in the oil phase.
Once the double emulsion is created, the oil from the membrane needs to be removed, leaving only the lipid bilayer (Fig. 2.b). This removal can be spontaneous or triggered, depending on the characteristics of the molecules involved.
Multivesicular microfluidic vesicles follow a similar production process. The main difference is that the smaller vesicles that will be encapsulated into the larger ones are added to the inner phase.
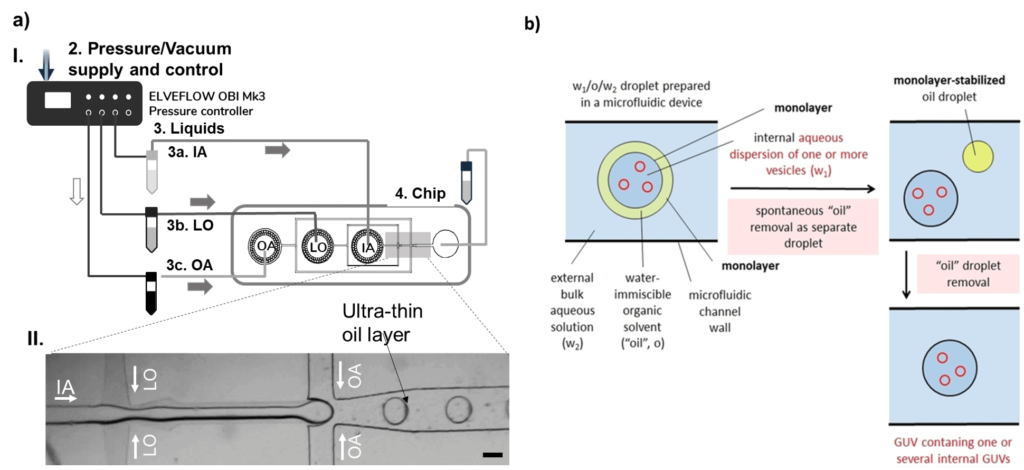
Multivesicular microfluidic vesicles for drug delivery
Liposomes have been approved and used as drug delivery systems since 1995 [10]. They improve the therapeutic index of drugs such as anticancer doxorubicin and daunorubicin but are also known to have limitations in retaining small-molecule drugs once in systemic circulation [6].
It is believed that the early release is caused by interactions of the lipid bilayer with enzymes in the blood, causing premature membrane degradation.
The hierarchical structure of multivesicular vesicles can help solve this problem by providing an extra layer of protection. Also, each vesicle can be tuned to answer a particular trigger, increasing formulation flexibility.
One approved formulation, the DepoFoam, takes advantage of this property [11]. For example, it has been shown that it allows a much higher dose of non-opioid pain relievers without worsening side effects [12].
Traditional emulsification methods produce it. However, manufacturing challenges resulted in the discontinuation of some products that used DepoFoam as a carrier. A more mature microfluidic technology could potentially address these challenges.
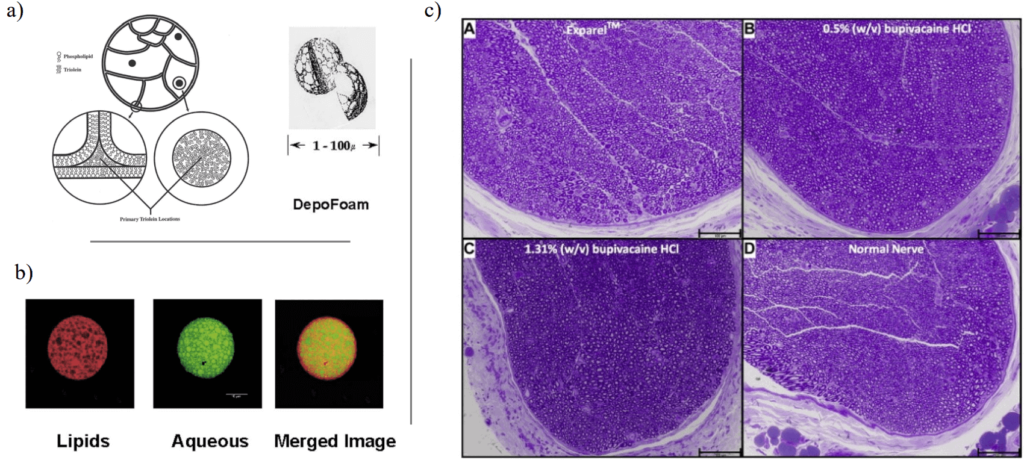
Multivesicular microfluidic vesicles artificial-cell like systems
Multivesicular microfluidic vesicles closely resemble the configuration of living cells, making them suitable models for artificial-cell-like systems [13].
Applications in this field range from confined chemical reaction systems to control complex chemical reactions in compartments to mimicking living cells, in which even cell division has been reproduced [14].
A great example of the use of multivesicular microfluidic vesicles as artificial-cell-like systems is the work of Deng et al., 2016. They reported an artificial cell with a “nucleus” capable of RNA transcription. The synthetic cell was a multivesicular vesicle produced with microfluidic glass capillaries.
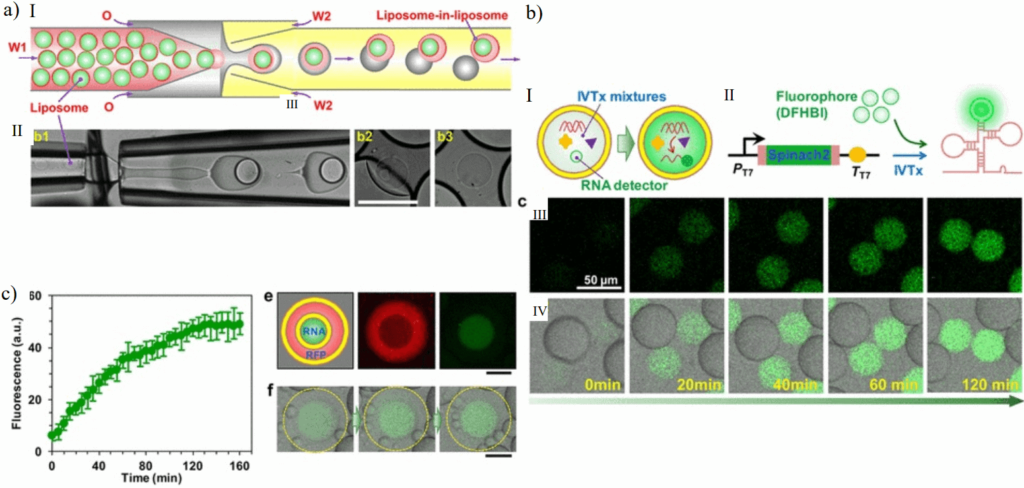
Conclusion and Outlook
Multivesicular microfluidic vesicles are promising tools for advancing drug delivery systems and artificial-cell-like systems research. For drug delivery, they provide extra protection for liposomes in the bloodstream while allowing for fine-tuning of the system for better release, taking into consideration the properties of the drug and administration route.
Artificial-cell-like systems closely resemble living cells, allowing the study of confined reaction systems and more complex cellular behaviors, such as division and RNA transcription.
Microfluidics has advantages over traditional emulsification methods due to improved monodispersity of liposomal populations, increasing the reproducibility of the experiments. It also provides higher control over encapsulation and compartment composition.
These characteristics can improve results across the discussed applications and will be particularly important for the pharmaceutical industry once the technology reaches the industry scale.
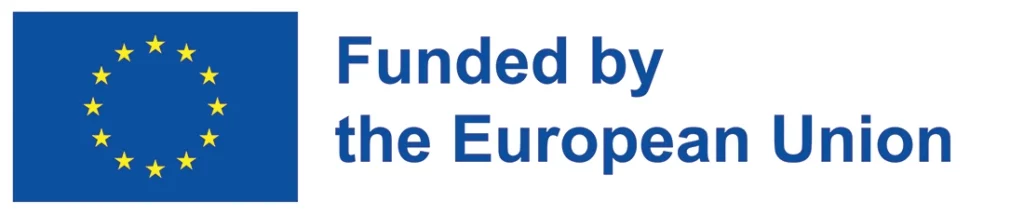
Review done thanks to the support of the Protomet H2020-MSCA-ITN-2018-Action “Innovative Training Networks”, Grant agreement number: 813873
Author: Camila Betterelli Giuliano, PhD
Contact:
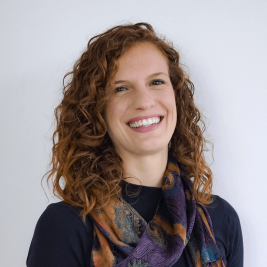
References
- P. Walde and S. Ichikawa, ‘Enzymes inside lipid vesicles: Preparation, reactivity and applications’, Biomolecular Engineering, vol. 18, no. 4. Elsevier, pp. 143–177, 31-Oct-2001.
- P. Walde, Encycopedia of Nanoscience and Nanotechnology, Vol 9. Stevenson Ranch: American Scientific Publishers, 2004.
- P. Walde, ‘Building artificial cells and protocell models: Experimental approaches with lipid vesicles’, BioEssays, vol. 32, no. 4. Bioessays, pp. 296–303, Apr-2010.
- U. Bulbake, S. Doppalapudi, N. Kommineni, and W. Khan, ‘Liposomal formulations in clinical use: An updated review’, Pharmaceutics, vol. 9, no. 2, pp. 1–33, Jun. 2017.
- N.-N. Deng, M. Yelleswarapu, L. Zheng, and W. T. S. Huck, ‘Microfluidic Assembly of Monodisperse Vesosomes as Artificial Cell Models’, J. Am. Chem. Soc., vol. 139, no. 2, pp. 587–590, Jan. 2017.
- E. T. Kisak, B. Coldren, C. A. Evans, C. Boyer, and J. A. Zasadzinski, ‘The Vesosome-A Multicompartment Drug Delivery Vehicle’, 2004.
- N. Nuti, P. E. Verboket, and P. S. Dittrich, ‘Multivesicular droplets: A cell model system to study compartmentalised biochemical reactions’, Lab Chip, vol. 17, no. 18, pp. 3112–3119, Sep. 2017.
- S. Deshpande, Y. Caspi, A. E. C. Meijering, and C. Dekker, ‘Octanol-assisted liposome assembly on chip’, Nat. Commun., vol. 7, pp. 1–9, 2016.
- T. Trantidou, Y. Elani, and O. Ces, ‘Microfluidic generation of double emulsions as multiphase compartmentalised cell-like systems’, IET Conf. Publ., vol. 2016, no. CP702, 2016.
- U. Bulbake, S. Doppalapudi, N. Kommineni, and W. Khan, ‘Liposomal formulations in clinical use: An updated review’, Pharmaceutics, vol. 9, no. 2, pp. 1–33, 2017.
- Q. Ye, J. Asherman, M. Stevenson, E. Brownson, and N. V. Katre, ‘DepoFoam(TM) technology: A vehicle for controlled delivery of protein and peptide drugs’, in Journal of Controlled Release, 2000, vol. 64, no. 1–3, pp. 155–166.
- B. M. Ilfeld, N. Malhotra, T. J. Furnish, M. C. Donohue, and S. J. Madison, ‘Liposomal bupivacaine as a single-injection peripheral nerve block: A dose-response study’, Anesth. Analg., vol. 117, no. 5, pp. 1248–1256, Nov. 2013.
- J. W. Hindley et al., ‘Light-triggered enzymatic reactions in nested vesicle reactors’, Nat. Commun., vol. 9, no. 1, pp. 1–6, Dec. 2018.
- W. Zong, S. Ma, X. Zhang, X. Wang, Q. Li, and X. Han, ‘A Fissionable Artificial Eukaryote-like Cell Model’, J. Am. Chem. Soc., vol. 139, no. 29, pp. 9955–9960, Jul. 2017.