Fungus identification and research using microfluidics: a review
Published on August 4th 2020
We can start with the fungi definition to understand fungus identification and research using microfluidics.
A fungus is any member of the eukaryotic organism group, including microorganisms like molds and yeasts. Collectively, all fungi make a separate kingdom, “Fungi,” which is distinct from plants and animals.
One of the main characteristics that separate the fungi from other kingdoms is the presence of chitin in their cell wall. Fungi are distributed worldwide and diverse in their habitat (figure 1).
They are everywhere, from air to soil to water and even ice. Fungi are common pathogens in clinical practices and crop plants, as they also play an essential role in maintaining the ecological balance.
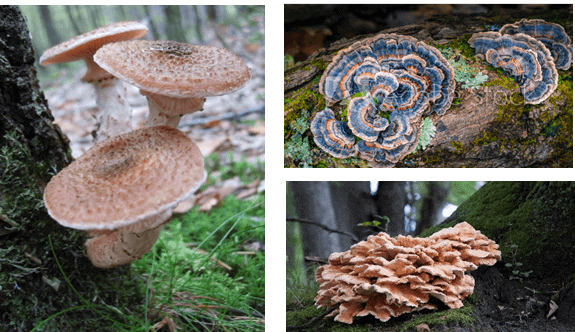
Introduction to fungus identification and research
They also play a pivotal role in the microbial industry and biomedical research. The conventional procedures for fungal identification are laborious, time-consuming, expensive, and unable to realize single-cell analysis.
In this review, we described the fungal detection based on morphology or molecular basis and recent advances in microfluidic devices for fungal identification.
The applications of fungi research based on microfluidic chips were finally summarized, which aimed to provide new ideas and directions for future research. Developing a real-time continuous analytical platform for pathogen detection is of great scientific importance for achieving better disease control and prevention.
Conventional approaches for fungal detection
A key to the classification and taxonomy of an organism is its correct identification. The accurate identification of pathogenic fungi is vital for quarantine purposes. Also, they serve as a foundation for developing efficient disease management strategies [1]. The detection methods of fungi can be roughly divided into two categories: based on morphology and based on molecular biology.
Morphological detection of fungi
Fungi possess unique morphological characteristics which can be used to distinguish them with the naked eye. Hence, fungi have been previously identified based on their morphological characteristics, including macroscopic and microscopic characteristics. This video shows a colony of fungi growing in a petri dish.
Morphological identification includes direct examination under a microscope and staining. However, it becomes very difficult to differentiate closely related pathogenic species based on morphological characteristics, as most species share similar culture characteristics, including colony and spore appearance [2].
For example, the clear differentiation between the large-spored Alternaria species cannot be made based on cultural and morphological characteristics (figure 2) as there are comparatively limited morphological features with narrow differences, which results in overlapping and incorrect identification of the isolates [3].
Furthermore, morphological characteristics are also influenced by cultural conditions [4]. So, staining is required for fungi that are difficult to distinguish under the microscope. Common fungal staining methods include Gram staining, Giemsa staining, PAS staining, Gomori methenamine silver staining, and so on.

Due to the limitations by conventional techniques for fungi identification (which are only focused on the detection and identification of fungi by morphological characteristics), the rapid development of micro-imaging technology and microfluidic chips makes it possible to observe fungi germination and growth in real time.
Spore germination into actively growing mycelium is a vital process for the survival and pathogenesis of fungi. Nevertheless, the molecular mechanisms controlling the germination and growth of fungi are poorly understood partly because few tools exist to evaluate the processes.
Molecular detection of fungi
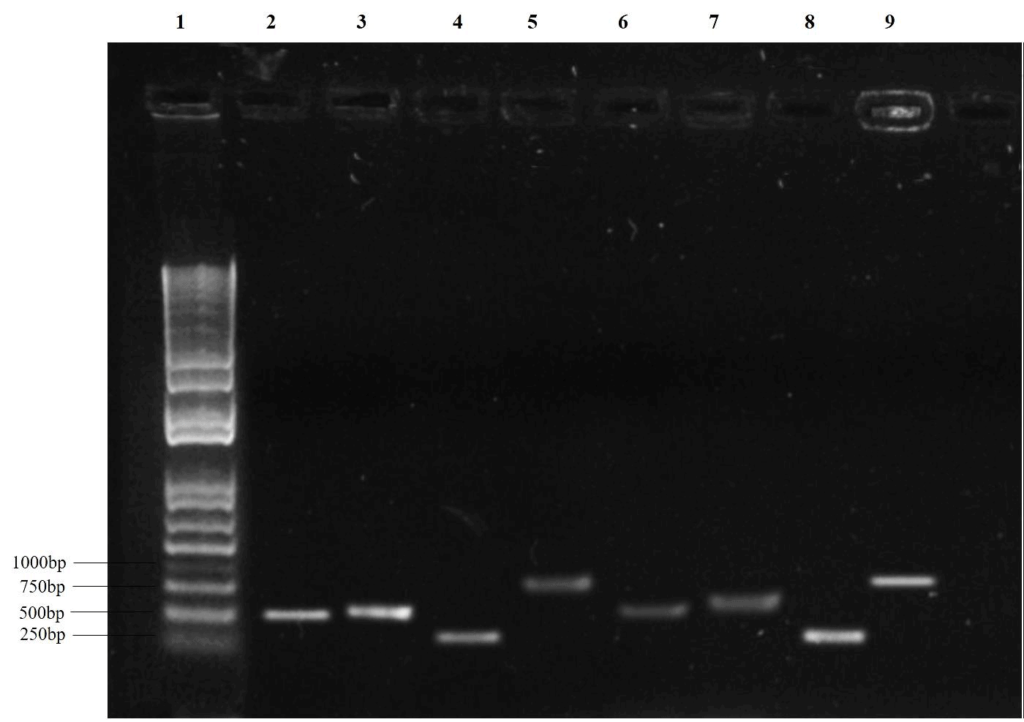
In the past two decades, several techniques have been developed for molecular identification of the organisms [5]. Polymerase chain reaction (PCR) with specific oligonucleotide primers have been extensively applied to identify pathogens [6, 7]. Universal primers, such as those amplifying the internal transcribed spacer region (ITS) region of rDNA, are used to identify fungi [8].
The nuclear rDNA, including small and large subunits that are variable at the family, genus, or species level, proved to be an ideal target for specific PCR primers [8]. The ITS regions have been broadly used in molecular phylogeny and taxonomical studies as they can be readily amplified using small quantities of DNA [9, 10, 11]. The translation elongation factor 1 alpha (TEF-1-α) loci is highly conserved in translation processes.
The TEF-1α gene illustrates the genetic diversity, examines the intra- and inter-specific variation, and analyzes the phylogenetic diversity among eukaryotic organisms [12, 13, 14] (figure 3).
Microfluidic approaches for fungal identification
Over the last two decades, channel-based microfluidics, using microfluidic flow controllers, has appeared with robust approaches for confining and manipulating single cells within physical or chemical boundaries while sustaining an in-vivo environment.
Each species has particular genetic information or specific antigens different from others, which allows the identification of fungi on microfluidic chips, not limited to a morphological/molecular basis.
Barkal et al. [15] fabricated a device comprising a microfluidic platform and an image analysis pipeline to study the germination process of fungal spores (figure 4). This platform enabled highly quantitative, statistically meticulous single-cell spore and population-based assessments of germination in high resolution and the assay utilized to test conditions needed for C. neoformans spore germination.
The results depicted the potential of the microfluidic device applied to chemical and genetic approaches, offering a deep understanding of the germination process at the molecular level. It also provided a tested and ready platform for identifying and assessing new potential antifungal drugs targeting germination.
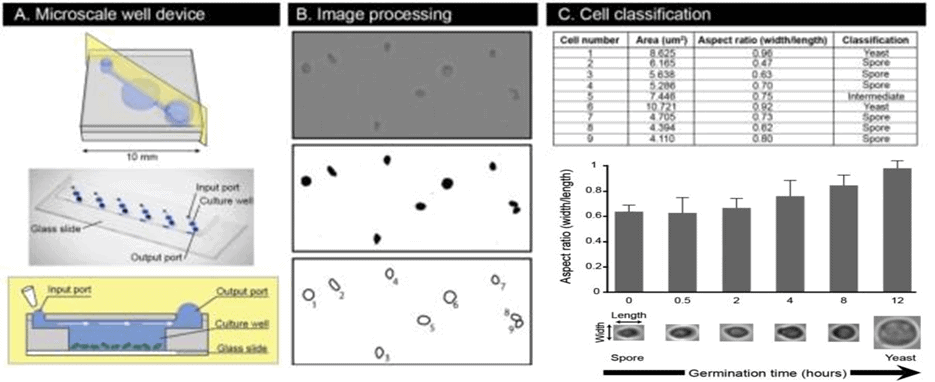
With the advancement in microfluidic chip technology, the microfluidic techniques have been combined with fungal detection and identification techniques to achieve higher efficiency and accuracy. Soares et al. [16] fabricated an ultrafast single-step fluorescence-based competitive immunoassay, employing nanoporous agarose beads functionalized with protein A or G confined in a microfluidic chamber to detect aflatoxin B1.
A fluorescence microscope was used to observe the results; the fluorescence signal decreased when the sample contained a target object. Aflatoxin B1 was detected at one ng/mL after a 2-min assay time, among the fastest reported in the literature. This chip can be used as a powerful tool for screening mycotoxins.
Lee et al. [17] developed a simple polydimethylsiloxane (PDMS)– based microfluidic device (Figure 5) comprising 2D spiral microchannels. This device was applied for real-time imaging of single-nucleus behavior in Neurospora crassa. Time-lapse microscopy permits comprehensive phenotyping of fungi.
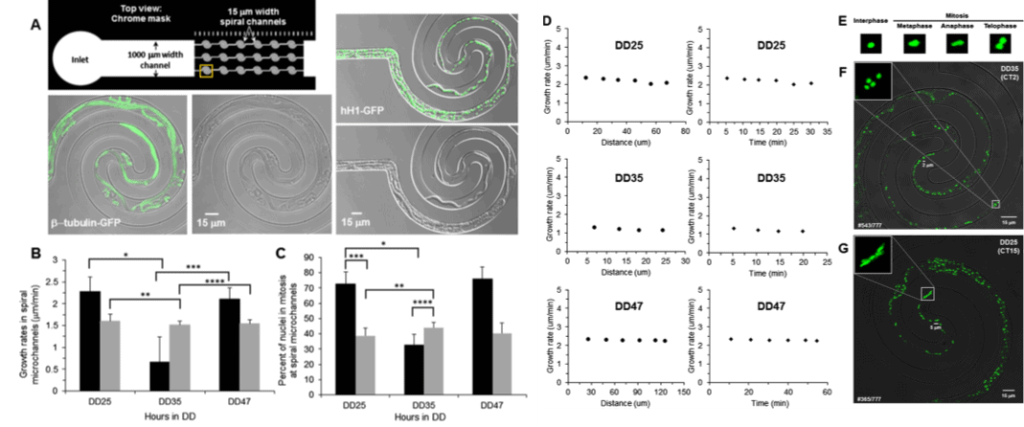
The microfluidic platform offers a significant technical advance in detecting microbial DNA in clinical specimens. With the development of various mycotoxin-specific monoclonal antibodies and the immuno-affinity column for single and multiple mycotoxins, the advantages of high throughput, high sensitivity, skill, and rapidity of the microfluidic chip will be more prominent in the synchronous detection of the fungi. Marshall et al. [18] fabricated a PDMS microfluidic device to study growth, germination, and sporulation in Fusarium virguliforme, a causal agent of sudden death syndrome in soybeans.
This kind of microfluidic chip device with quantitative time-lapse microscopic photography can be applied to screen compound peptides and identify fungal or antifungal agents to combat devastating plant pathogens.
The chip can identify candidate target genes for host-induced gene silencing in fungal pathogens for generating novel disease resistance in crop plants. Recently, combined with some unknown capture and sorting methods, the real-time observation of the germination and proliferation of single fungal spores can be examined more effectively.
Here is a protocol for performing antifungal screening on a chip using droplet-based microfluidics.
Discover how to perform single-spore encapsulation.
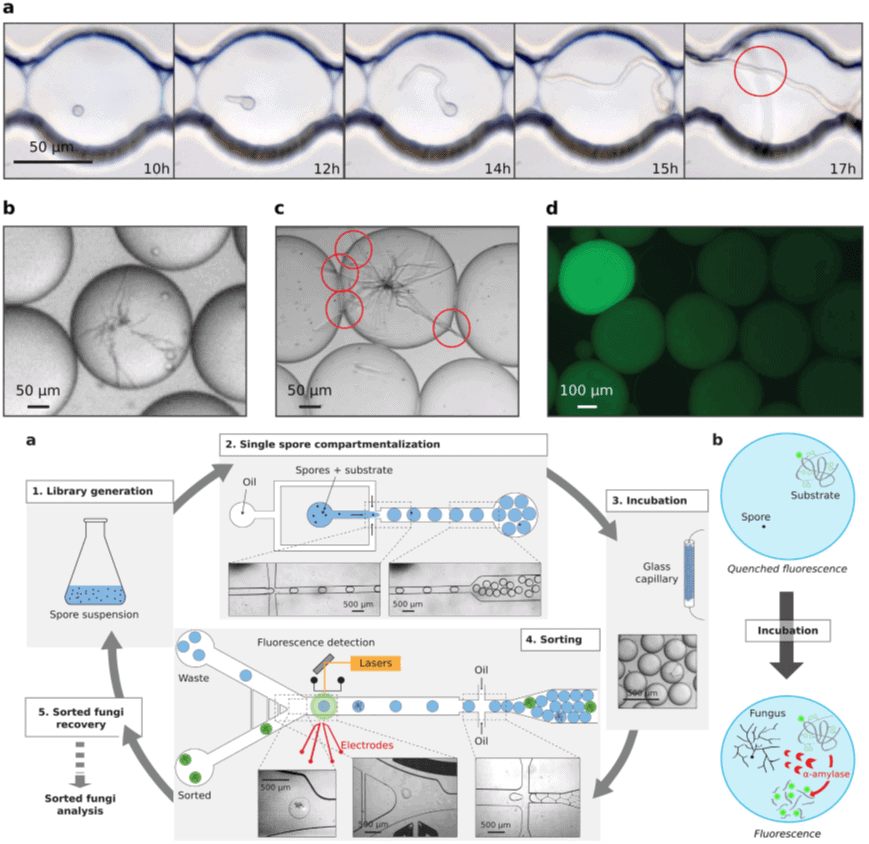
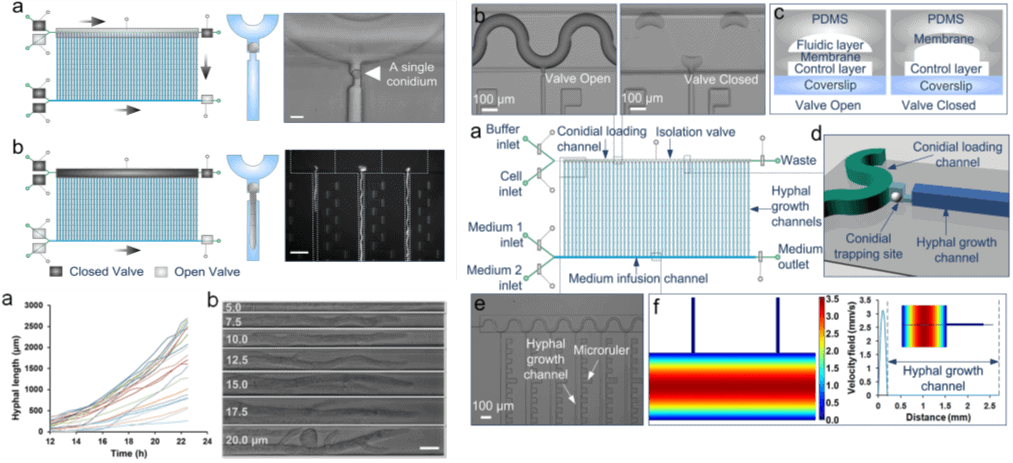
Species of Candida frequently cause life-threatening infections in neonates, transplant and intensive care unit patients, and others with compromised host defenses. The successful management of systemic candidiasis depends upon early, rapid diagnosis.
Schell et al. [21] developed a digital microfluidic real-time PCR platform to detect DNA of Candida albicans in blood; eleven and nine of 16 specimens from individual patients with culture-proven candidemia tested positive for C. albicans DNA by conventional and microfluidic real-time PCR, respectively, for a combined sensitivity of 94%.
Richter [22] et al. describe a novel combination of contactless dielectric microsensors and microfluidics to promote biofilm formation for fungal analysis. The chip (Figure 8) consists of a PDMS system bonded to a glass wafer containing the electrodes, while external heating and pumping stations control temperature and fluid flow.
Fungi are cultured in microchambers of microfluidic chips, which allows the creation of biological niches by providing relevant fluidic conditions and maintaining stable temperature profiles over long periods. The different dynamic behavior of fungal biofilm is revealed by stimulating different concentrations of antifungal drugs.
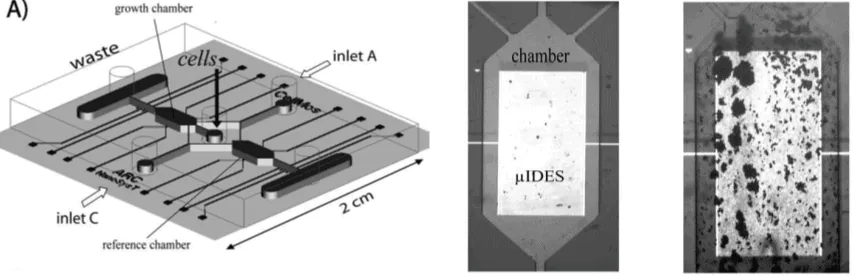
Hanson [23] developed a PDMS microfluidic maze chip (figure 9) to evaluate the growth behavior and optimality of fungal space-searching algorithms by monitoring hyphal growth directionality in the micro-confined network in real-time; the study suggests that even simple microorganisms have developed adequate strategies to solve nontrivial geometrical problems.
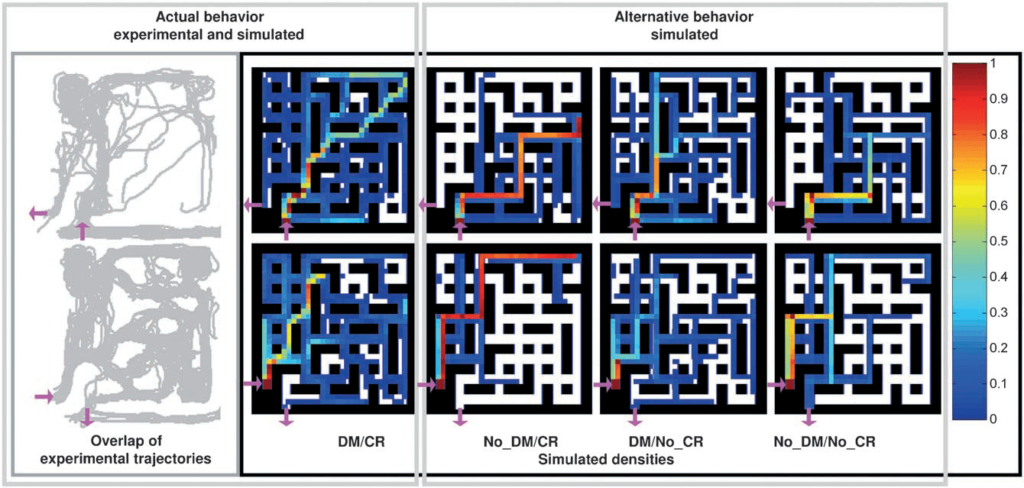
Conclusion and future perspectives for fungus identification and research
The review summarizes recent advances in microfluidic devices for fungus identification and research, including the materials detection methods and their applications. Detection methods based on different principles, such as optical, electrochemical, and biological technology, have the potential to detect fungi more efficiently and sensitively than traditional methods.
Microfluidic technology provides a complete quantitative system for microbial research, which not only solves a series of problems such as fungal growth, chemotaxis, and cell cycle but also can simulate the natural environment to research the state of microbes, population dynamics, and genetic evolution under environmental pressure [24]. This emerging technology exhibits great potential for exploring the complex world of fungi.
At the same time, several challenges still need to be resolved. One critical challenge in the future is to elucidate the metabolism interaction of fungi and evaluate the effect of drugs on diseases caused by fungi. Recently, benefiting from the development of microfluidic chips and mass spectrometry, chip-mass spectrometry (Chip-MS) has become an ambient platform for cell analysis [25].
In perspective, Chip-MS will also contribute to studying microbial metabolism, clinical diagnosis and treatment of diseases, and drug screening. In addition, biomaterials can participate in cell analysis by enhancing metabolite accumulation, realizing single-cell analysis, and enhancing sensitivity in Chip-MS analysis [26]. Overall, it is believed that microbiology collides with microfluidic technology and will contribute more to the research on microorganisms in the future.
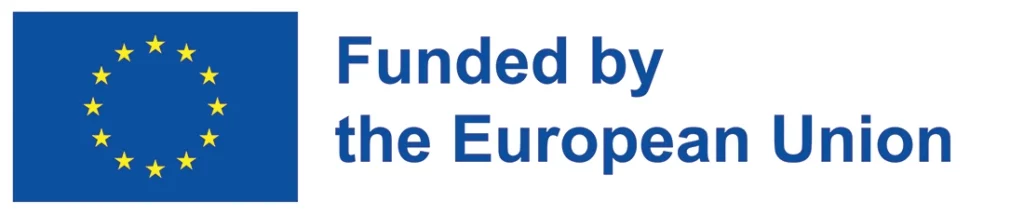
Review done thanks to the support of the MAHT-FunSST H2020-MSCA-IF-2018, Grant agreement number: 843162
Author: Sehrish Iftikhar, PhD
Post-doctoral fellow
Contact:
Partnership[at]microfluidic.fr
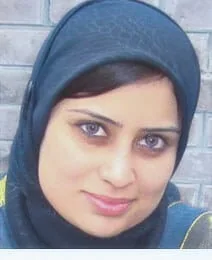
References
- Rossman, A.Y. and Palm-Hernández, M.E., 2008. Systematics of plant pathogenic fungi: why it matters. Plant Disease, 92(10), pp.1376-1386. Manamgoda, D.S., Cai, L., McKenzie, E.H., Crous, P.W., Madrid, H., Chukeatirote, E., Shivas, R.G., Tan, Y.P. and Hyde, K.D., 2012. A phylogenetic and taxonomic re-evaluation of the Bipolaris-Cochliobolus-Curvularia complex. Fungal Diversity, 56(1), pp.131-144.
- Manamgoda, D.S., Cai, L., McKenzie, E.H., Crous, P.W., Madrid, H., Chukeatirote, E., Shivas, R.G., Tan, Y.P. and Hyde, K.D., 2012. A phylogenetic and taxonomic re-evaluation of the Bipolaris-Cochliobolus-Curvularia complex. Fungal Diversity, 56(1), pp.131-144.
- Anees, M., Tronsmo, A., Edel-Hermann, V., Hjeljord, L.G., Héraud, C. and Steinberg, C., 2010. Characterization of field isolates of Trichoderma antagonistic against Rhizoctonia solani. Fungal biology, 114(9), pp.691-701.
- Diguta, C.F., Vincent, B., Guilloux-Benatier, M., Alexandre, H. and Rousseaux, S., 2011. PCR ITS-RFLP: a useful method for identifying filamentous fungi isolates on grapes. Food microbiology, 28(6), pp.1145-1154.
- Paplomatas, E.J., 2004. Molecular diagnostics for soilborne fungal pathogens. Phytopathologia Mediterranea, 43(2), pp.213-220.
- Zitnick-Anderson, K.K. and Nelson Jr, B.D., 2015. Identification and pathogenicity of Pythium on soybean in North Dakota. Plant disease, 99(1), pp.31-38.
- Lakshman, D.K., Jambhulkar, P.P., Singh, V., Sharma, P. and Mitra, A., 2016. Molecular identification, genetic diversity, population genetics and genomics of Rhizoctonia solani. Perspectives of Plant Pathology in genomic era, pp.55-89.
- White, T.J., Bruns, T., Lee, S.J.W.T. and Taylor, J.L., 1990. Amplification and direct sequencing of fungal ribosomal RNA genes for phylogenetics. PCR protocols: a guide to methods and applications, 18(1), pp.315-322.
- Voigt, K., Cigelnik, E. and O’donnell, K., 1999. Phylogeny and PCR identification of clinically important Zygomycetes based on nuclear ribosomal-DNA sequence data. Journal of Clinical Microbiology, 37(12), pp.3957-3964.
- Iwen, P.C., Hinrichs, S.H. and Rupp, M.E., 2002. Utilization of the internal transcribed spacer regions as molecular targets to detect and identify human fungal pathogens. Medical mycology, 40(1), pp.87-109.
- Schoch, C.L., Seifert, K.A., Huhndorf, S., Robert, V., Spouge, J.L., Levesque, C.A., Chen, W. and Fungal Barcoding Consortium, 2012. Nuclear ribosomal internal transcribed spacer (ITS) region as a universal DNA barcode marker for Fungi. Proceedings of the National Academy of Sciences, 109(16), pp.6241-6246.
- Zarrin, M., Ganj, F. and Faramarzi, S., 2016. Development of a polymerase chain reaction-restriction fragment length polymorphism method for identification of the Fusarium genus using the transcription elongation factor-1α gene. Biomedical reports, 5(6), pp.705-708.
- Pearson, K.A., Taylor, A.F.S., Fuchs, R.M.E. and Woodward, S., 2016. Characterisation and pathogenicity of Fusarium taxa isolated from ragwort (Jacobaea vulgaris) roots. Fungal ecology, 20, pp.186-192.
- Klopfenstein, N.B., Stewart, J.E., Ota, Y., Hanna, J.W., Richardson, B.A., Ross-Davis, A.L., Elías-Román, R.D., Korhonen, K., Keča, N., Iturritxa, E. and Alvarado-Rosales, D., 2017. Insights into the phylogeny of Northern Hemisphere Armillaria: Neighbor-net and Bayesian analyses of translation elongation factor 1-α gene sequences. Mycologia, 109(1), pp.75-91.
- Barkal, L.J., Walsh, N.M., Botts, M.R., Beebe, D.J. and Hull, C.M., 2016. Leveraging a high resolution microfluidic assay reveals insights into pathogenic fungal spore germination. Integrative Biology, 8(5), pp.603-615.
- Soares, R.R.G., Santos, D.R., Pinto, I.F., Azevedo, A.M., Chu, V., Aires-Barros, M.R. and Conde, J.P., 2016. Point-of-use ultrafast single-step detection of food contaminants: a novel microfluidic fluorescence-based immunoassay with integrated photodetection. Procedia Engineering, 168, pp.329-332.
- Lee, K.K., Labiscsak, L., Ahn, C.H. and Hong, C.I., 2016. Spiral-based microfluidic device for long-term time course imaging of Neurospora crassa with single nucleus resolution. Fungal Genetics and Biology, 94, pp.11-14.
- Marshall, J., Qiao, X., Baumbach, J., Xie, J., Dong, L. and Bhattacharyya, M.K., 2017. Microfluidic device enabled quantitative time-lapse microscopic-photography for phenotyping vegetative and reproductive phases in Fusarium virguliforme, which is pathogenic to soybean. Scientific reports, 7, p.44365.
- Beneyton, T., Wijaya, I.P.M., Postros, P., Najah, M., Leblond, P., Couvent, A., Mayot, E., Griffiths, A.D. and Drevelle, A., 2016. High-throughput screening of filamentous fungi using nanoliter-range droplet-based microfluidics. Scientific reports, 6, p.27223.
- Geng, T., Bredeweg, E.L., Szymanski, C.J., Liu, B., Baker, S.E., Orr, G., Evans, J.E. and Kelly, R.T., 2015. Compartmentalized microchannel array for high-throughput analysis of single cell polarized growth and dynamics. Scientific reports, 5, p.16111.
- Schell, W.A., Benton, J.L., Smith, P.B., Poore, M., Rouse, J.L., Boles, D.J., Johnson, M.D., Alexander, B.D., Pamula, V.K., Eckhardt, A.E. and Pollack, M.G., 2012. Evaluation of a digital microfluidic real-time PCR platform to detect DNA of Candida albicans in blood. European journal of clinical microbiology & infectious diseases, 31(9), pp.2237-2245.
- Richter, L., Stepper, C., Mak, A., Reinthaler, A., Heer, R., Kast, M., Brückl, H. and Ertl, P., 2007. Development of a microfluidic biochip for online monitoring of fungal biofilm dynamics. Lab on a Chip, 7(12), pp.1723-1731.
- Hanson, K.L., Nicolau Jr, D.V., Filipponi, L., Wang, L., Lee, A.P. and Nicolau, D.V., 2006. Fungi use efficient algorithms for the exploration of microfluidic networks. small, 2(10), pp.1212-1220.
- Son, K., Brumley, D.R. and Stocker, R., 2015. Live from under the lens: Exploring microbial motility with dynamic imaging and microfluidics. Nature Reviews Microbiology, 13(12), p.761.
- Mao, S., Li, W., Zhang, Q., Zhang, W., Huang, Q. and Lin, J.M., 2018. Cell analysis on chip-mass spectrometry. TrAC Trends in Analytical Chemistry.
- Ning, R., Zhuang, Q. and Lin, J.M., 2018. Biomaterial-based microfluidics for cell culture and analysis. In Cell Analysis on Microfluidics (pp. 181-224). Springer, Singapore.